
диафрагмированные волноводные фильтры / 337a4942-28bc-49a2-b0c4-8266f12b2d20
.pdf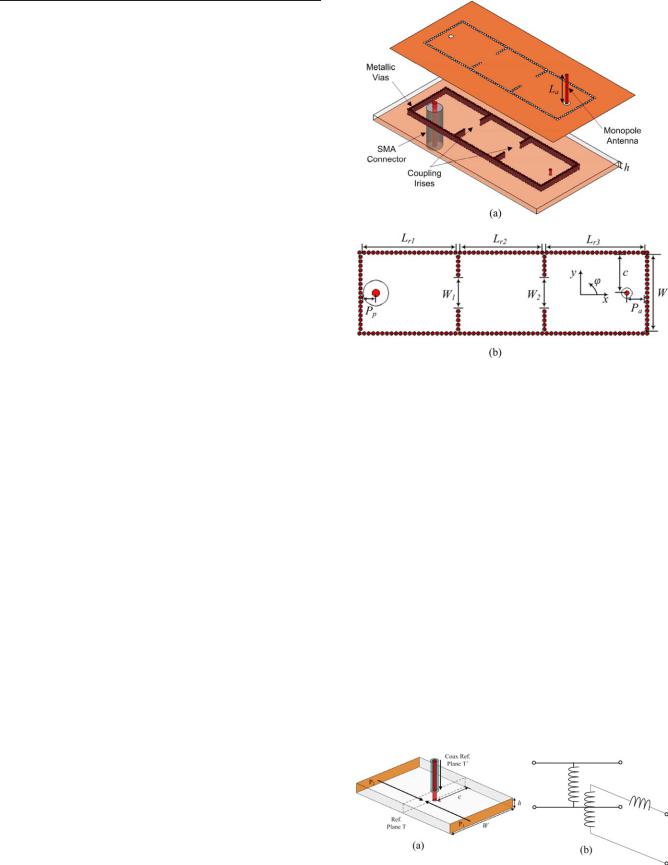
INTEGRATION OF 3D HIGH-Q FILTERS WITH MONOPOLE ANTENNAS
Yazid Yusuf1 and Xun Gong2
1Triquint Semiconductors, Apopka, FL 32703; Corresponding author: yazidn@gmail.com
2Antenna, RF and Microwave Integrated (ARMI) System Laboratory, Department of Electrical Engineering and Computer Science, University of Central Florida (UCF), Orlando, FL 32816
Received 1 August 2013
ABSTRACT: Seamless integration of 3D cavity filters with monopole antennas is presented. The typical 50-X transition between the filter and antenna is eliminated to form a compact and efficient RF front end. A three-pole cavity filter integrated with a monopole antenna is demonstrated at X band. The center frequency and fractional bandwidth of the filter/antenna system are 10.07 GHz and 6.5%, respectively. The efficiency of the entire filter/antenna system is shown to be 93% in fullwave simulations. A prototype filter/antenna system is fabricated and measured. Excellent agreement between the simulated and measured results is observed. The integration approach presented herein can be used for other types of wire antennas depending on the application. VC
2014 Wiley Periodicals, Inc. Microwave Opt Technol Lett 56:921–925, 2014; View this article online at wileyonlinelibrary.com. DOI 10.1002/ mop.28221
Key words: bandpass filter; cavity resonator; monopole antenna; substrate integrated waveguide
1.INTRODUCTION
As the demand for high-performance transceivers increases, methods of implementing compact and efficient RF front ends are actively being investigated. Filters and antennas are critical components in many communications and radar systems. Typically, filters and antennas are cascaded through 50-X connections, whereby filters are utilized to reject out-of-band noise and interference while transmitting in-band signals. High-quality (Q)-factor resonator filters based on waveguide cavities [1], dielectric resonators [2], and substrate integrated waveguides [3] are preferred over planar structures due to their lower insertion loss, a key factor that helps improve the system efficiency and sensitivity.
In [4], the authors presented a synthesis technique to integrate high-Q 3D filters with slot antennas. By codesigning the filter and antenna into an inseparable unit, the 50-X transition between,the otherwise separate structures is eliminated, resulting in more compact and efficient systems. In this article, we extend the technique in [4] to the integration of 3D cavity filters with monopole antennas, which provide vertical polarization and omnidirectional radiation patterns. The efficiency of the entire filter/antenna system is shown to be as high as 93% at X band. A prototype filter/antenna is designed, fabricated, and measured. Excellent agreement between measured and simulated results is observed.
2.FILTER/ANTENNA SYNTHESIS
The schematic of a three-pole Chebyshev bandpass cavity filter integrated with a monopole antenna is shown in Figure 1. The cavities are realized using closely spaced metallic vias, whose separation is small enough compared to the wavelength to minimize radiation loss from the cavities [5]. The internal coupling between cavities is through irises in the common sidewalls. The external coupling to the first cavity is from a short-ended SMA connector. A monopole antenna is electrically connected to the
Figure 1 (a) Skew and (b) top view of a three-pole cavity filter integrated with a monopole antenna. (W 513; Pp 52.3; W1 55.5; W2 55.6; Lr1 515.5; Lr2 513.6; Lr3 516.5; Pa 53.2, c 56.5; La 57; h 51.57) Dimensions are in mm. [Color figure can be viewed in the online issue, which is available at wileyonlinelibrary.com]
bottom side of the third cavity through a circular opening on its top side. It should be noted that this connection is not standard 50 X. To the authors’ best knowledge, such integration between cavity filters and monopole antennas has not been reported. Due to its relatively wide bandwidth, the monopole antenna behaves as an equivalent load as seen by the filter, if designed correctly.
The response of the filter/antenna will exhibit the same filtering function, if the resonant frequency of each resonator, the internal coupling coefficient kij, and the external quality factor Qext are identical to a reference filter. In this regard, the monopole antenna should provide the same external coupling level as the port of the reference filter. In addition, the frequency loading effect of the antenna, which is generally different from that of the port, should be appropriately compensated for. Section 2.1. presents the equivalent circuit model for this integrated monopole antenna and the design curves for Qext. The filter/ antenna synthesis procedure is described in section 2.2.
Figure 2 (a) Schematic of a waveguide excited by a coaxial probe and (b) its equivalent circuit. (W 513, c 56.5, h 51.57) Dimensions are in mm. [Color figure can be viewed in the online issue, which is available at wileyonlinelibrary.com]
DOI 10.1002/mop |
MICROWAVE AND OPTICAL TECHNOLOGY LETTERS / Vol. 56, No. 4, April 2014 |
921 |

Figure 3 (a) Resonator/antenna structure, (b) equivalent circuit of the resonator/antenna, (c) simplified equivalent circuit, and (d) equivalent series RLC circuit. [Color figure can be viewed in the online issue, which is available at wileyonlinelibrary.com]
2.1. Modeling the Cavity and Monopole Antenna
We first consider a waveguide which is fed by a coaxial probe as shown in Figure 2(a). This structure was studied by Harrington for thin feeding probes [6]. Its equivalent circuit is shown in Figure 2(b). This equivalent circuit uses an ideal transformer with 1:n turns ratio and a reactance of Xp from the probe. Using a stationary treatment and assuming the characteristic impedance of the waveguide Z0 51 X, the turns ratio n is given by [6]:
|
w |
W |
kh |
|
W |
|
|
n2 |
5Z |
2h |
tan ðkhÞ |
|
2sin 2 |
pc |
(1) |
|
|
|
|
where h and W are the waveguide height and width, respectively; c is the distance between the center of the probe and the sidewall of the waveguide; k is the wave number in the dielectrically loaded waveguide; and Zw is the TE10 wave impedance.
The structure consisting of the monopole, the coupling probe, and the third cavity of the filter, referred to as the resonator/ antenna is illustrated in Figure 3(a). It can be obtained by connecting a monopole antenna at the reference plane T0 of the structure in Figure 2(a) and replacing one of the ports with a
short-circuiting wall. The equivalent circuit of the resonator/ antenna is shown in Figure 3(b), in which Za represents the impedance of the monopole antenna. The monopole antenna coupled through the probe can be modeled as a shunt impedance, Zshunt, as shown in Figure 3(c), which is given by:
1 |
jXp1Za |
|
Zshunt 5n2 |
(2) |
in which the turns ratio n modifies Zshunt and can be used to control the coupling between the cavity resonator and monopole antenna. In this article, the freedom in choosing n is not exploited in order to reduce the number of design parameters.
In order to extract Zshunt, the structure in Figure 4 is first simulated using Ansoft High Frequency Structure Simulator (HFSS). The monopole antenna length La is chosen to be 7 mm and the substrate is 1.57-mm-thick Rogers RT/Duroid 5880 (er 52.2 and tan d 5 0.0009). The diameters of the probe (same as the monopole antenna) and the hole on the top of the cavity are chosen as 0.635 and 1.27 mm, respectively. Zshunt can be extracted as the Z12 of two-port network at the reference plane T and plotted in Figure 4, in which Z0 5 1 X is used as the
Figure 4 Zshunt for a monopole antenna of length La 57 mm. [Color figure can be viewed in the online issue, which is available at wileyonlinelibrary. com]
Figure 5 Input impedance of the cavity resonator with a monopole antenna. [Color figure can be viewed in the online issue, which is available at wileyonlinelibrary.com]
922 |
MICROWAVE AND OPTICAL TECHNOLOGY LETTERS / Vol. 56, No. 4, April 2014 |
DOI 10.1002/mop |

Figure 6 Extracted Qext vs. monopole antenna position Pa for antenna lengths La 56, 7, and 8 mm. [Color figure can be viewed in the online issue, which is available at wileyonlinelibrary.com]
characteristic impedance of the waveguide. Using the equivalent circuit in Figure 3(c), the input impedance of the resonator/ antenna Zin is calculated and shown in Figure 5. It should be noted that for any Pa value, Lr30 is always adjusted to achieve a resonant frequency f0 5 10 GHz. It is apparent from Figure 5 that the structure behaves like a series resistor-inductor-capacitor (RLC) resonator [Fig. 4(d)] around the resonant frequency. Particularly, Pa and Lr30 values used to generate Figure 5 are 3.2 and 17.8 mm, respectively. To verify the accuracy of the equivalent circuit in Figure 3(c), the resonator/antenna structure is also simulated in HFSS, which produces almost identical curves as the equivalent circuit.
The element values of the series RLC resonator are extracted using:
L3 |
5 |
1 |
|
dðIm ðZin ÞÞ |
f 5f0 |
(3) |
|
|
|
||||||
|
|
4p |
df |
|
|
||
|
C35 |
1 |
|
(4) |
|||
|
|
|
|||||
|
ð2pf0Þ2La |
|
|||||
|
|
|
|
|
|
|
R35Zin ðf0Þ |
(5) |
R3 can be divided into two parts, namely Rext and Ro3 that represent the radiation loss and the combined dielectric and metallic losses, respectively. By simulating the structure [Fig. 3(a)] with/without setting the dielectric and metallic losses to zero, Rext and Ro3 can be extracted. Qext, which corresponds to the coupling between the resonator and antenna, can then be calculated using:
Qext 5 |
2p f0L3 |
(6) |
|
Rext |
|||
|
|
The design curves for Qext corresponding to different combinations of Pa and La are presented in Figure 6. The close agreement between HFSS simulations and equivalent circuit model is apparent. Therefore, to reduce the design time, only several simulations with a parameter sweep on La are necessary in HFSS. Then, the extracted Zshunt is used in the equivalent circuit [Fig. 3(c)] to generate these design curves. From Figure 9, it is observed that there are many different La/Pa combinations which could be used to achieve a certain Qext.
2.2. Filter/Antenna Design
A 7.2% fractional bandwidth three-pole Chebyshev filter/ antenna is designed at 10 GHz on a 1.57-mm-thick Rogers RT/ Duroid 5880 substrate. The design parameters are:
k125k2350:046 |
(7) |
Qext;15Qext;2524:1 |
(8) |
Using Figure 6, La and Pa are chosen to be 7 and 3.2 mm, |
|
respectively, in order to obtain Qext 5 24.1. Again, it |
is noted |
that this choice of La/Pa combination represents only one possible design. Lr30 is set to 17.8 mm to make the resonator/antenna resonate at f0 510 GHz. At this stage, L3, C3, Ro3, and Rext can be found using (3)–(5).
Using the equivalent circuit of the resonator/antenna in Figure 3(c), the equivalent circuit of the entire filter/antenna system can be constructed as shown in Figure 7. K inverters are used to represent the external coupling from the input port to the first
Figure 7 Equivalent circuit of the three-pole integrated filter/antenna system (K01 52.87 X, K12 50.182 X, K23 50.19 X, Lr10 5Lr20 516.1, Pa 53.2, Lr30 517.8). Dimensions are in mm
Figure 8 Lumped element equivalent |
circuit of the filter/antenna. (K01 52.87 X; K12 50.182 X; K23 50.19 X; L1 5L2 563.2 pH; C1 5C2 54 pF; |
Ro1 5Ro2 55.67 mX; L3 569 pH; C3 5 |
3.67 pF; Ro3 56.2 mX; Rext 50.178 X |
DOI 10.1002/mop |
MICROWAVE AND OPTICAL TECHNOLOGY LETTERS / Vol. 56, No. 4, April 2014 |
923 |

Figure 9 Simulated and measured responses of the three-pole integrated filter with a monopole antenna. [Color figure can be viewed in the online issue, which is available at wileyonlinelibrary.com]
resonator (K01) and the internal coupling between the cavity resonators (K12 and K23). The cavity resonators are modeled by kg/ 2 transmission lines at 10 GHz with length Lr10 and Lr20. The equivalent circuit of the filter/antenna can also be represented using lumped elements as shown in Figure 8. The purpose of developing the lumped element equivalent circuit is to derive the K inverter values. L1 and L2 are obtained by equating the slope of the reactance, kg/2 transmission line resonator to that of a series LC circuit at the resonant frequency f0 5 10 GHz. L1 and L2 are given by:
p2er f0 |
|
L15L25Z0 c02b2 |
(9) |
where c0 is the speed of light in free space and b is the propagation constant of the waveguide evaluated at f0. The coupling coefficient between resonators i and j is given by [7]:
Kij
kij5 p (10) 2pf0 LiLj
As L3 calculated from (3) is generally different from L1 and L2, an impedance inverter K23 different from K12 is needed to obtain k12 5k23 50.046. The filter/antenna system with the design parameters shown in (7) and (8) is synthesized, using the equivalent circuit in Figure 8, in which the obtained circuit parameters are listed. The resistances Ro1, Ro2, and Ro3 account for the unloaded Q factor of the cavity resonators which is approximately 700.
Using the approach in [8], Pp, W1, and W2 needed to realize the impedance inverters K01, K12, and K23 are determined. The physical lengths of the resonators Lr1 and Lr2 are obtained by adjusting Lr10 and Lr20 to account for the negative transmission line lengths of the impedance inverters. The dimensions of the designed filter/antenna are listed in Figure 1(b). The monopole antenna is mounted on a 100 3 100 mm2 ground plane. The simulated return loss and gain of the filter/antenna using HFSS are presented in Figure 9. S11 is less than 212 dB within the passband. It should be noted that this S11 level can be further reduced by designing a smaller Qext. The gain versus frequency in the azimuth plane at u 5 90 clearly demonstrates the filtering function of the filter/antenna. Similar curves are found at all other radiation directions. The simulated maximum gain and directivity, which occur at 64o zenith angle, are 4.1 and 4.42 dBi, respectively, implying 93% efficiency by taking the ratio of the two.
3. RESULTS AND DISCUSSION
A prototype integrated filter/antenna is fabricated and measured. The measured S11 agrees well with simulation results as shown in Figure 9. The 0.7% upshift in frequency is due to fabrication tolerances. The measured filter/antenna bandwidth of 6.5% is slightly smaller than the simulated 7.2%. The gain of the filter/antenna system versus frequency is measured in an anechoic chamber and is
Figure 10 Simulated and measured radiation patterns of the integrated filter with a monopole antenna in (a) H plane and (b) E plane at the center frequency. [Color figure can be viewed in the online issue, which is available at wileyonlinelibrary.com]
924 |
MICROWAVE AND OPTICAL TECHNOLOGY LETTERS / Vol. 56, No. 4, April 2014 |
DOI 10.1002/mop |

plotted against the simulation results as shown in Figure 9. It is noted that the gain is measured in the azimuth plane at u 590 to directly compare with simulations. At 10 GHz, the measured gain is shown to 20.58 dBi, which is close to the simulated gain of 20.35 dBi.
The measured and simulated radiation patterns in both H plane and E plane (u 590 ) at the center frequency are shown in Figure 10. The small discrepancy between the simulated and measured radiation patterns is due to the scattering from the antenna mounting structure and cables. The small ripple in the H plane radiation pattern is caused by the diffraction from the finite-size ground plane.
4.CONCLUSION
A synthesis approach to integrate high-Q 3D filters with monopole antennas was presented. The integrated filter/antennas preserve the filtering and radiating characteristics with reduced size and improved efficiency. The measurement results from the prototype verified the synthesis approach.
ACKNOWLEDGMENT
The authors acknowledge the generous donation of substrate materials from Rogers Corporation.
REFERENCES
1.A.E. Atia and A.E. Williams, Narrow-bandpass waveguide filters, IEEE Trans Microwave Theory Tech 20 (1972), 258–265.
2.C. Wang and K.A. Zaki, Dielectric resonators and filters, IEEE Microwave Mag 8 (2007), 115–127.
3.Y.L. Zhang, W. Hong, K. Wu, J.X. Chen, H.J. Tang, Novel substrate integrated waveguide cavity filter with defected ground structure, IEEE Trans Microwave Theory Tech 53 (2005), 1280–1287.
4.Y. Yusuf and X. Gong, Compact low-loss integration of high-Q 3-D filters with highly efficient antennas, IEEE Trans Microwave Theory Tech 59 (2011), 857–865.
5.D. Deslandes and K. Wu, Single-substrate integration technique of planar circuits and waveguide filters, IEEE Trans Microwave Theory Tech 51 (2003), 593–596.
6.R.F. Harrington, Time-harmonic electromagnetic fields, Wiley, New York, NY, 2001.
7.G.L. Matthaei, L. Young, and E.M.T. Jones, Microwave filters, impedance-matching networks, and coupling structures, Artech House, Norwood, MA, 1980.
8.R.J. Cameron, C.M. Kudsia, and R.R. Mansour, Microwave filters for communication systems: fundamentals, design, and applications, Chapter 14, Wiley, Hoboken, NJ, 2007.
VC 2014 Wiley Periodicals, Inc.
A CPW-FED CIRCULAR SLOT UWB ANTENNA WITH WLAN BAND AND X-BAND FILTERING CHARACTERISTICS USING HYBRID RESONATORS
Yingsong Li,1 Wenxing Li,1 and Wenhua Yu2
1College of Information and Communications Engineering, Harbin Engineering University, Harbin, Heilongjiang 150001, China; Corresponding author: liwenxing@hrbeu.edu.cn
22COMU, Inc., Fairfax, VA 22030
Received 8 August 2013
ABSTRACT: A compact coplanar waveguide (CPW)-fed circular slot ultrawideband (UWB) antenna with dual-band filtering characteristic is presented in this article. The dual-band filtering characteristic is achieved by inserting an arc-rectangle-shaped stepped impedance
resonator in the circular ring radiation patch and etching a spiral stepped impedance resonator in the CPW-fed transmission line. The numerical and experimental results demonstrate that the proposed antenna can operate on a frequency band between 2.7 and 12 GHz with voltage standing wave ratio less than 2, except for that two filtering bands between 4.8 and 6 GHz and between 7.6 and 8.6 GHz are used to prevent the potential interference from WLAN band and X-band. In addition, the proposed antenna has a compact size and omnidirectional radiation pattern, which is suitable for UWB communication applications. VC 2014 Wiley Periodicals, Inc. Microwave Opt Technol Lett 56:925–929, 2014; View this article online at wileyonlinelibrary.com. DOI 10.1002/mop.28242
Key words: ultrawideband antenna; dual-band filtering antenna; stepped impedance resonator; T-shaped stub
1. INTRODUCTION
The ultrawideband (UWB) technology has received considerable attention due to its high-speed data rate and low power consumption, and has been widely studying in the recent years. One of the key components in an UWB system is UWB antenna that has been investigating in engineering applications and academic research [1]. Plenty of UWB antennas in a compact size, wide bandwidth, and omnidirectional radiation patterns have been proposed to meet the requirements of UWB systems [1–3]. However, many narrow frequency bands have been using in different areas for a long time, such as IEEE 802.11a WLAN systems operating from 5.15 to 5.825 GHz, X-band for satellite communications from 7.9 to 8.4 GHz, and ITU 8-GHz communication systems from 7.725 to 8.275 GHz, which might have potential interferences with UWB systems. In some cases, narrow band stop filter is used to trickle the interferences between UWB systems and narrow band systems, which will increase complexity and cost of UWB systems. Thus, designing UWB antennas with filtering characteristic may be one of the essential methods toward an efficient and inexpensive device to get rid of unexpected signals. Recently, several methods have been proposed to design UWB antennas with filtering characteristics including etching C-shaped slot [4, 5], H-shaped slot [6], halfcircle slots [7], and circular slots [8] on the radiation patch or ground plane. However, these slots may have electromagnetic wave leaking, and in turn, deteriorate the radiation patterns. Several band filtering UWB antennas are proposed to improve antenna performance by using stubs [9, 10] and etching slots and using parasitic strips [11–13]. Resonators [14, 15] are also used to design dual-band filtering UWB antennas at the same time.
In this article, a compact coplanar waveguide (CPW)-fed circular slot UWB antenna with dual-band filtering characteristic is proposed and its performance is verified numerically and experimentally. By inserting arc-rectangle-shaped stepped impedance resonator (ARS-SIR) in ring radiation patch and etching spiral stepped impedance resonator (SSIR) in CPW-fed transmission line, two filtering bands at WLAN band and X-band are achieved, respectively. It is observed from both numerical and experimental results that the proposed dual-band filtering UWB antenna can operate on a frequency band between 2.7 and 12 GHz with voltage standing wave ratio (VSWR) less than 2. Two filtering frequency bands between 4.8 and 6 GHz and between 7.6 and 8.6 GHz are used to sieve the unexpected signals from WLAN band and X-band. In addition, the proposed dual filtering bands can be adjusted to meet various interference suppression applications, and the antenna has a wide bandwidth and
DOI 10.1002/mop |
MICROWAVE AND OPTICAL TECHNOLOGY LETTERS / Vol. 56, No. 4, April 2014 |
925 |
Copyright of Microwave & Optical Technology Letters is the property of John Wiley & Sons, Inc. and its content may not be copied or emailed to multiple sites or posted to a listserv without the copyright holder's express written permission. However, users may print, download, or email articles for individual use.