
диафрагмированные волноводные фильтры / ae0c402f-2c79-480d-8c04-4f7684308d47
.pdf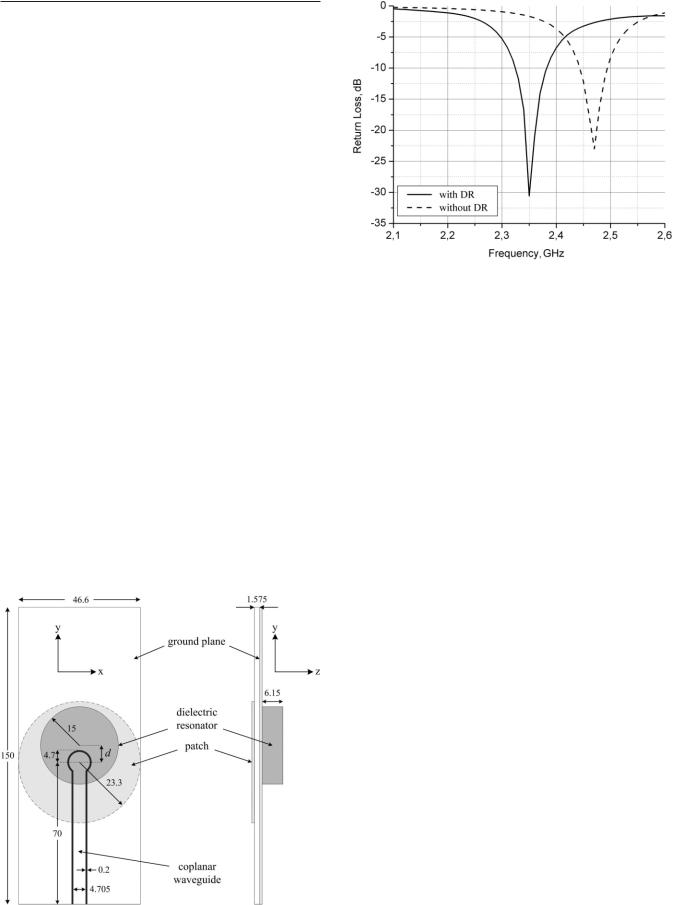
CPW-FED OMNIDIRECTIONAL HYBRID MICROSTRIP/DIELECTRIC RESONATOR ANTENNA
Alexandre Perron,1 Tayeb A. Denidni,1 and Abdel R. Sebak2
1 Institut National de la Recherche Scientifique (INRS), Montreal, QC, Canada; Corresponding author: perrona@emt.inrs.ca
2 Concordia University, Montreal, QC, Canada
Received 5 February 2008
ABSTRACT: In this letter, we present a new antenna configuration in which a coplanar waveguide (CPW) is used to feed a cylindrical dielectric resonator (DR) and a subjacent circular patch to generate an omnidirectional radiation pattern. A first prototype, designed to resonate near 2.45 GHz, shows that an impedance bandwidth of 6.3% is achievable using a single layer of substrate. © 2008 Wiley Periodicals, Inc. Microwave Opt Technol Lett 50: 2699 –2701, 2008; Published online in Wiley InterScience (www.interscience.wiley.com). DOI 10.1002/mop. 23734
Key words: coplanar waveguides; dielectric resonator antennas; microstrip antennas
1. INTRODUCTION
Omnidirectional antennas, such as dipole antennas, are commonly used in wireless local area networks (WLANs) and other mobile communication systems. Using a dipole is often a simple and cost-effective way of generating an omnidirectional radiation pattern. Unfortunately, these antennas can become too bulky for some applications, especially those requiring a higher gain, that is, an antenna array. In this perspective, a back-to-back rectangular microstrip antenna fed by a coplanar waveguide (CPW) has been proposed in [1]. For this configuration, two microstrip patches are etched on the opposite sides of two stacked substrate layers sandwiching the ground plane and the CPW feed. Unfortunately, the measured bandwidth is limited to less to 1.7%, which is not enough to cover the whole ISM 2.4 –2.5 GHz frequency band. In
Figure 1 Geometry of the proposed antenna (dimensions in mm)
Figure 2 Simulated return loss of the antenna (with and without the DR)
this letter, we propose a new antenna configuration with a larger impedance bandwidth that is based on a single layer of substrate.
2. ANTENNA DESIGN
Figure 1 shows the topology of the proposed antenna. In fact, it is a hybrid composed of a microstrip antenna and a dielectric resonator antenna (DRA). DRAs are becoming increasingly popular, because they offer several advantages over conventional antennas. Amongst others, they exhibit high radiation efficiency due to the absence of conductor or surface wave losses, and they are less susceptible to tolerance errors, especially at millimeter wave frequencies [2]. Finally, as for microstrip antennas, different structures can be used for feeding, including probes, microstrip lines, and CPWs [3-5]. In our case, using a CPW was of particular interest, because the dielectric resonator (DR) could be placed directly on top of the coplanar line on the ground plane’s side. This way, a microstrip patch could be etched on the bottom of the substrate to produce the needed backward radiation.
Both radiating elements are excited via a small loop at the end of the CPW, as shown in Figure 1. This loop acts as a short dipole, having the same effect as a probe entering the DR to excite the HEM11 mode (maximum at broadside). The same coupling mechanism applies to the microstrip patch, exciting the TM011 mode (also having its maximum at broadside). Note that the patch is centered on the loop, but that the DR is at an offset (d in Fig. 1); this is mainly for impedance matching purposes.
3. SIMULATION RESULTS
Ansoft HFSS was used to optimize the antenna. The final simulated dimensions (in mm) are shown in Figure 1. The optimal value of d was found to be 8.9 mm. When d was less than 8.9 mm, the antenna’s port was overcoupled and vice versa. The dimensions of the 50 CPW feed were calculated using the software LineCalc. The radius of the microstrip patch and the dimensions of the DR were set for resonance at 2.45 GHz. Note that the width of the substrate matches the diameter of the patch; this allows uniform radiation in the x–z plane to achieve omnidirectional radiation. Finally, the relative dielectric constant of the substrate and the resonator are 2.2 and 35.5, respectively.
Figure 2 shows the simulated return loss of the proposed
DOI 10.1002/mop |
MICROWAVE AND OPTICAL TECHNOLOGY LETTERS / Vol. 50, No. 10, October 2008 |
2699 |

Figure 3 Simulated radiation patterns of the antenna at 2.35 GHz
antenna. The impedance matching is very good and the resulting bandwidth (VSWR 2) is about 60 MHz (2.55%). The return loss of the microstrip antenna, without DR, is also shown in this figure. As we can see, adding the DR has the effect of downshifting the resonant frequency without significantly altering the bandwidth, but, as illustrated in Figure 3, an omnidirectional radiation pattern is generated. Both E and H-plane patterns are normalized to 0 dB. As expected, the maxima of the E-plane are at 0 and 180°, and the whole pattern looks like the number “eight.” Simulations have shown that the small dips at 30°, 150°, 210°, and 330° are the consequence of using a narrow substrate. The simulated maximum gain is about 2.75 dB on each side, and the radiation efficiency is near 100%. The H-plane pattern is almost perfectly omnidirectional. In fact, the gain values on the sides (at 90° and 270°) are less than 1.5 dB down from the maximum values (about 2.75 dB). In both cases, the cross polarization is not negligible, but still within an acceptable range, that is, 17 dB or less.
4. EXPERIMENTAL RESULTS
A prototype was built using substrate RT/Duroid 5880 ( r 2.2, thickness 1.575 mm, and tan 0.0009). In the prototyping
Figure 4 Measured return loss of the prototype (with and without the DR)
Figure 5 Measured radiation patterns of the prototype at 2.37 GHz
phase, we wanted to see if it would be possible to extend the impedance bandwidth of the antenna by separating, to some extent, the resonant frequencies of the radiating elements. Therefore, all the values in Figure 1 were kept as listed except for the height of the DR and the offset d. The final value of the former was 6.4 mm; 0.5 mm for the later.
An Agilent 8722ES Network Analyzer was used to measure the S11 parameter. The results are shown in Figure 4. As expected, the resulting impedance bandwidth is wider (6.3%), and the resonant frequency is slightly downshifted as the DR is added to the antenna. Impedance matching was done by adjusting the parameter d, compromising for a wider bandwidth instead of a perfectly adapted port.
The fabricated prototype was placed in an automated anechoic chamber for far-field radiation pattern measurements. The results are shown in Figure 5 and are in good agreement with the simulation results. Indeed, the E-plane is eight-shaped and the H-plane pattern is nearly circular. Once again, the cross-polarization levels are significant. Nonetheless, the omnidirectional behavior of the proposed antenna is evident. Measurements (not shown here) were also made at the boundaries of the impedance bandwidth, and it was confirmed that the radiation characteristics of the antenna are consistent over the 150 MHz bandwidth.
5. CONCLUSION
An omnidirectional CPW-fed hybrid antenna has been designed and fabricated. It has been shown that a 6.3% impedance bandwidth is achievable with a single layer of substrate. This low profile antenna could be used for WLAN applications or as part of an array. The design process is very straightforward, and the same principle has already been successfully applied to a similar antenna comprised a rectangular DRA and a rectangular microstrip patch.
REFERENCES
1.H. Iwasaki, A back-to-back rectangular-patch antenna fed by a CPW, IEEE Trans Antennas Propag 46 (1998), 527–1530.
2.A. Petosa, A. Ittipiboon, Y.M.M. Antar, D. Roscoe, and M. Cuhaci, Recent advances in dielectric-resonator antenna technology, IEEE Antennas Propag Mag 40 (1998), 35– 48.
3.G.P. Junker, A.A. Kishk, A.W. Glisson, and D. Kajfez, Input impedance of dielectric resonator antennas excited by a coaxial probe, IEEE Trans Antennas Propag 42 (1994), 960 –966.
2700 |
MICROWAVE AND OPTICAL TECHNOLOGY LETTERS / Vol. 50, No. 10, October 2008 |
DOI 10.1002/mop |

4. R.A. Kranenburg and S.A. Long, Microstrip transmission line excitation of dielectric resonator antennas, Electron Lett 24 (1988), 1156 –1157.
5.R.A. Kranenburg and S.A. Long, Coplanar waveguide excitation of dielectric resonator antennas, IEEE Trans Antennas Propag (1991), 119-122.
© 2008 Wiley Periodicals, Inc.
MINIATURIZED MICROSTRIP FILTERS AND DIPLEXERS FOR WIRELESS COMMUNICATION SYSTEMS
Damir Zayniyev,1 Djuradj Budimir,1 and George Zouganelis2
1 Wireless Communications Research Group, Department of Electronic Systems, University of Westminster, 115 New Cavendish Street, London, W1W 6UW, United Kingdom; Corresponding author: d.budmir@wmin.ac.uk
2 Department of Materials Science and Engineering, Nagoya Institute of Technology, Gokiso-cho, Showa-Ku, Nagoya 466-8555, Japan
Received 5 February 2008
ABSTRACT: A highly miniaturized microstrip diplexer composed of pseudointerdigital quasi-elliptical bandpass filters is proposed. Compactness of diplexer is achieved through the combination of the bandpass filters, which does not require matching network. The proposed microstrip diplexer, having a size of 30.7 5.6 mm at a center frequency of 2.7 GHz at channel 1 and 3.8 GHz at channel 2 is designed, fabricated, and tested. Experimental results of the fabricated microstrip diplexer are in very good agreement with the simulated results. © 2008 Wiley Periodicals, Inc. Microwave Opt Technol Lett 50: 2701–2702, 2008; Published online in Wiley InterScience (www.interscience.wiley. com). DOI 10.1002/mop.23733
Key words: microstrip diplexer; bandpass filters; interdigital filters; hairpin filters; pseudointerdigital filter
1. INTRODUCTION
Broadband wireless access communications systems is a rapidly expanding market. Such systems commonly use filters and diplexers in microwave and millimeter-wave transceivers as channel separators [1–10]. Hence, there is an increasing demand for low cost, and compact size filters and diplexers. Diplexers are threeport devices, which are commonly used after multiband or wide band antenna, take two or more frequencies in input port, and separate them to two output ports.
Conventional design procedure of the microwave diplexer/ multiplexer consists of two steps. First step is the design of microwave filters, which are usually bandpass/bandstop structures [1] sometimes combined with lowpass/highpass filters [2]. Second step is the combination of developed filters using matching networks [3]. Usually, different types of T-junctions [4] are used. The main requirement for any multiplexer is a high isolation between filters and low VCWR on the common port. Very often, a connection of different filters to common port causes interaction and
Figure 2 Simulated S-parameters of the microstrip diplexer
degradation of initial transfer characteristics of filters and additional reconfiguration of filters is required.
In this article, super compact microstrip diplexers for WiMAX was designed using novel super compact microstrip pseudo-inter- digital quasi-elliptical bandpass filters. The proposed diplexer has a passband center frequency 2.7 GHz for the channel 1 and 3.8 GHz passband center frequency for channel 2.
2. DIPLEXER DESIGN
To develop a diplexer, a second bandpass filter with pass band at 3.6 GHz was designed using the same procedure as described earlier. Connection of both filters to common port using traditional T-junctions [5] caused introduction of additional losses and degradation of transmission of one of the filters. The easiest way to avoid this was to redesign one of the filters in such way to have both of them connected to the same feeding line. The configuration of bandpass filter was altered to move the feeding line to the top. This combination also reduces the total size of diplexer, which is 30.7 5.6 mm. The layout of designed diplexer is shown in Figure 1.
Figure 1 Layout of the proposed microstrip diplexer |
Figure 3 Measured S-parameters of the microstrip diplexer |
DOI 10.1002/mop |
MICROWAVE AND OPTICAL TECHNOLOGY LETTERS / Vol. 50, No. 10, October 2008 |
2701 |