
диафрагмированные волноводные фильтры / 528e2820-65ef-41ca-973f-5e2e224ab323
.pdf
Confidential manuscript submitted to replace this text with name of AGU journal
Compact E-plane Waveguide Filter with Multiple Stopbands
Marija Mrvić, Milka Potrebić, and Dejan Tošić
School of Electrical Engineering, University of Belgrade, Serbia
Corresponding author: Milka Potrebić (milka_potrebic@etf.rs)
Key Points:
E-plane dual-band and triple-band bandstop waveguide filters using quarter-wave resonators exhibit independent control of the stopbands
Miniaturization is achieved by grounding the resonators for different stopbands to the opposite waveguide walls
Novel equivalent electrical circuit of the multi-band bandstop waveguide filter provides fast filter design and optimization
Abstract
This paper presents a novel miniaturized E-plane multi-band bandstop waveguide filter. Quarter-wave resonators (QWRs) are printed on a thin dielectric substrate to form an insert that is positioned in the E-plane of a rectangular waveguide. Stopbands are designed by simple adjustment of length of the QWRs. QWRs for different stopbands are spaced on the insert to eliminate the unwanted coupling between them. Proposed design exhibits independent tuning of the center frequency and bandwidth of each stopband. Miniaturization is achieved by attaching the QWRs for different stopbands to the opposite waveguide walls. As a proof of concept, dual-band and triple-band E-plane waveguide bandstop filters with independent control of designed stopbands (ICDS) are designed. Further size reduction is made by changing dimensions of the dual-band filter, leading to the proposed ultra-compact (UC) filter when designed stopbands are not independently controllable. Next, influence of the individual parameters on the filter response is thoroughly investigated. Novel equivalent electrical circuit is presented for fast filter design. Equivalent circuit of the multi-band bandstop filter is a cascade of the equivalent networks of the single-band bandstop filters. Utilization of the proposed circuit enables design fine-tuning, thus reducing the number of repeated time and memory consuming three-dimensional electromagnetic (3D EM) simulations. To verify the proposed design, an E-plane dual-band ICDS bandstop waveguide filter is fabricated, operating at f01 = 9 GHz with fractional bandwidth (FBW) of 5.77% and f02 = 11 GHz with FBW of 5.27%. Measured results are in good agreement with the 3D EM simulation.
This article has been accepted for publication and undergone full peer review but has not been through the copyediting, typesetting, pagination and proofreading process which may lead to differences between this version and the Version of Record. Please cite this article as doi: 10.1002/2016RS006169
© 2016 American Geophysical Union. All rights reserved.

Confidential manuscript submitted to replace this text with name of AGU journal
1. Introduction
Permanent development of modern communications implies new design requirements to components operating at microwave and millimeter-wave frequencies. Microwave filters, as essential components in devices purposed for radars, mobile and satellite communications, are evolving significantly in order to satisfy the necessity of high performance, compact size and functionality at the same time on several independently controlled frequencies. According to the required applications, the design process starts with the filter specification and proceeds with several steps preceding the final stage, which is implementation of the desired filter [Lutovac et al., 2001]. Microwave filters can be implemented using different technologies. Design examples are proposed in planar technology [Canete Rebenaque et al., 2008], substrate integrated waveguide (SIW) technology [Coves et al., 2016], and waveguide technology [Martinez Mendoza et al., 2015; Stefanovski Pajović et al., 2015; Stefanovski et al., 2014].
Filters implemented in waveguide technology are actively exploited at microwave frequencies due to their capability to handle high power, have low loss and high quality factor (Q-factor) [Cameron et al., 2007]. An innovative example of multiplexer design using filters in waveguide technology is presented in [Pons Abenza et al., 2016], and compact diplexer using evanescent mode waveguide filter is presented in [Jin et al., 2016].
Split ring resonators (SRRs), along with their dual counterparts, complementary SRRs (CSRRs), emerged as an attractive type of resonator for compact waveguide filter design [Ortiz et al., 2005; Mrvić et al., 2014]. Their behavior can be modeled by using LC resonant circuits [Baena et al., 2005]. In the light of the E-plane waveguide filters, a very compact bandpass filter using half-wavelength C-shaped resonators and central folded stripline resonators is presented in [Jin et al., 2015], while a novel bandstop filter using quarter-wave resonators (QWRs) is proposed in [Stefanovski et al. 2015]. The use of an SRR array for the bandstop filter design is reported in [Jitha et al., 2006], outlining that bandwidth can be adjusted by the number of SRRs and position of the array within waveguide. Concerning E- plane waveguide filters featuring dual-band operation, novel design using metal inserts with coupled resonant slots is proposed for X-band applications [Lopez-Villaroya, 2012], while folded SRRs (FSRRs) are used for Ka and W-band filter design [Jin and Xue, 2015].
The aim of this paper is twofold. Firstly, to propose a novel compact E-plane waveguide filter with multiple stopbands. Single-band filter design based on QWRs, proposed in [Stefanovski et al., 2015], is extended to multi-band filter design. Bandstop filter is implemented using printed-circuit insert placed in the E-plane of the rectangular waveguide. Center frequency of the bandstop filter can be adjusted by changing the length of the used QWRs. For the multi-band bandstop filter design, QWRs for different stopbands are grounded to the same waveguide wall and spaced on the printed-circuit insert to avoid the unwanted coupling between them. Design example of the dual-band bandstop filter with independent control of the stopbands is presented. To reduce the overall size of the dual-band bandstop filter, QWRs for different stopbands are attached to the opposite waveguide walls, and shifted relative to each other to maintain the possibility of independent tuning. For the triple-band filter design, QWRs for specified stopband are shifted along the upper waveguide wall, while QWRs for other stopbands are mutually distanced and grounded to the lower waveguide wall. For applications where compact size is a primary concern, further progress in miniaturization is achieved by changing the dimensions of the initially designed dual-band bandstop filter. The proposed ultra-compact dual-band filter is designed using QWRs for different stopbands positioned one below another. Due to their proximity which causes the unwanted mutual coupling, control of the center frequencies and bandwidths is limited.
© 2016 American Geophysical Union. All rights reserved.

Confidential manuscript submitted to replace this text with name of AGU journal
Impact of the individual parameters on the filter response is extensively investigated and exposed.
Secondly, a novel equivalent electrical circuit of the multi-band bandstop filter with independently tunable stopbands is proposed. It is in the form of a cascade of the equivalent electrical networks of the single-band bandstop filters, and corresponds to the decomposed 3D filter structure. Equivalent circuit provides a fast filter design while less computational effort is required. In addition, optimization of the whole device can be accomplished by reducing the number of iterative 3D EM simulations.
For the design and analysis of the filter and its equivalent electrical circuit WIPL-D software is used (www.wipl-d.com). In order to validate the presented design, an E-plane dual-band bandstop filter is fabricated and tested. Measured response is in good agreement with the 3D EM simulation.
2. Bandstop Filter Design
This section is focused on the E-plane waveguide bandstop filter design. The first step in the process is the design of single quarter-wave resonator with resonant frequency f0.
Standard WR-90 waveguide of the 22.86 mm x 10.16 mm cross sectional area is used for the design of the QWR and filters considered throughout this research. It is assumed that the dominant mode of propagation is the transverse electric TE10 mode. Substrate selected for the printed-circuit insert implementation is fiberglass/PTFE resin laminate (TLE-95) from Taconic (www.taconic-add.com), with parameters: εr = 3, tanδ = 0.0028, substrate thickness h = 0.11176 mm and metallization thickness t = 0.0175 mm. The metal losses due to the skin effect and surface roughness are taken into account by setting the conductivity ζ = 20 MS/m.
2.1 Quarter-wave resonator and equivalent electrical circuit
3D model of the QWR is presented in Figure 1a. The dimensions of the presented QWR are d11=3.45 mm, d12=1.3 mm, c=0.2 mm, p=0.45 mm, l=0.5 mm for f0 = 9 GHz. Dimensions of the printed-circuit insert are ap = 22.86 mm and bp = 10.16 mm.
Figure 1.
An equivalent circuit model of the presented QWR is a simple parallel RLC resonant circuit shown in Figure 1b. Values of the R, L, C parameters were found from the following equations:
R 2 |
|
S11 jω0 |
|
Z0 |
|
1 |
|
S11 jω0 |
|
|
(1) |
|||||||||||
|
|
|
|
|||||||||||||||||||
L 2B |
|
S |
jω |
0 |
|
|
Z |
0 |
ω2 |
(2) |
||||||||||||
|
|
|||||||||||||||||||||
|
|
3dB |
|
11 |
|
|
|
|
|
|
0 |
|
|
|
||||||||
C 1 |
2B |
|
3dB |
|
S11 jω |
|
0 |
|
Z0 |
(3) |
||||||||||||
|
|
|
|
where ω0 denotes the angular frequency in (rad/s), B3dB is 3dB bandwidth (rad/s), S11(jω0) is the value of the S11 parameter at the considered resonant frequency. The impedance Z0 corresponds to the wave impedance of the waveguide for the chosen resonant frequency. In the considered case, for f0 = 9 GHz, Z0 = 550 Ω. Obtained values of the circuit parameters are
R=
7502
Ω, L
=
0.7506 nH, C = 0.4164 pF. The simulated frequency responses are compared for the equivalent circuit and the 3D EM model and shown in Figure 2. Good agreement between the obtained results is achieved in terms of the resonant frequency and bandwidth.
Figure 2.
© 2016 American Geophysical Union. All rights reserved.

Confidential manuscript submitted to replace this text with name of AGU journal
Quality factor (Q-factor) is an important parameter of a microwave resonator. Q- factor of the loaded resonator (QL) can be calculated from [see, for example, Goussetis et al.,
2011]: QL |
0 |
, where 0 |
and |
3dB |
are the resonant frequency of the resonator and the |
|
3dB |
||||||
|
|
|
|
|
3dB bandwidth, respectively. Q-factor of the unloaded resonator (QU) is obtained from the
equation: |
QU |
|
|
|
|
QL |
|
, where S21( 0 ) is the value of the S21 parameter at the resonant |
|
|
|
S21 0 |
|
||||
|
|
1 |
|
|
frequency. For the considered resonator, the Q-factors are QL=22.5 and QU = 175.34.
2.2. Bandstop filter using QWRs and equivalent electrical circuit
Second-order bandstop filter is designed with center frequency f0=9 GHz and FBW of 5.77%. QWRs are grounded to the lower waveguide wall, parallel to the waveguide sidewalls, as shown in Figure 3a. Dimensions of the used QWRs are given in subsection 2.1, and the distance between them is R1=8.5 mm. Equivalent electrical circuit of the considered filter, shown in Figure 3b, fully corresponds to the decomposed 3D EM model of the filter. The comparison of their frequency responses is shown in Figure 3c.
Figure 3.
Values of the equivalent circuit elements are determined throughout several steps. For the purpose of simplicity, losses haven’t been taken into consideration. QWRs are represented by LC resonators, with the magnetic (Lm) and electric (Cm) coupling between them. Values of L and C are calculated from equations (2) and (3). The impedances of ports are set to 550 Ω. For the waveguide section of length D1, its length is equal to the distance between middle parts of the QWRs in 3D EM model, so D1 ≈ d11/2+d11/2+R1. The length De1 is considered as the distance from the middle parts of the QWRs to the corresponding ends of the insert. Values of the coupling elements (Lm, Cm) are found from equation (4). This equation is given for the resonant frequencies (f1, f2) of the coupled QWRs. Numeric values of the resonant frequencies are determined for unloaded coupled resonators in the 3D EM model. In calculations, waveguide sections are represented by equivalent transmission lines. Therefore, a waveguide section of length D1 is represented by the equivalent transmission line of characteristic impedance Zc = 550 Ω, and electrical length θ = 1.60 rad at 9 GHz.
Equivalent circuit elements’ values are given in Table 1.
|
|
|
L Lm cot |
2 |
|
|
csc |
2 |
|
|
|
|
|
|||||||||
|
|
|
|
|
|
|
|
L Lm 4 C Cm Zc2 L Lm 4 C Cm Zc2 cos |
||||||||||||||
|
|
|
|
|
|
L Lm |
||||||||||||||||
f1 |
2 |
2 |
||||||||||||||||||||
|
|
|
|
|
|
|
|
|
|
|
|
|
|
|
|
|
|
|
|
|
|
|
|
|
|
|
|
|
|
|
|
|
4 C Cm L Lm Zc |
|
|
|
|
|
|
|
|||||
|
|
|
|
|
|
|
|
|
|
|
|
|
|
|
|
|
|
|||||
|
L L |
tan( |
|
|
|
|
|
|
|
|
|
|
|
|
cos |
2 |
|
|||||
|
2) |
|
|
L L |
|
4 C C |
Z 2 |
L L |
tan |
2 2 |
sign |
|||||||||||
f2 |
|
|
m |
|
|
|
|
m |
|
|
m |
c |
m |
|
|
|
|
|
||||
|
|
|
|
|
|
|
|
|
|
|
|
|
|
|
|
|
|
|
|
|
(4) |
|
|
|
|
|
|
|
|
|
|
2 C Cm L Lm Zc |
|
|
|
|
|
|
|
||||||
|
|
|
|
|
|
|
|
|
|
|
|
|
|
|
|
|
|
© 2016 American Geophysical Union. All rights reserved.

Confidential manuscript submitted to replace this text with name of AGU journal
3. Multi-Band Bandstop Waveguide Filters
In order to verify the design of the E-plane multi-band waveguide bandstop filter, filters with two and three stopbands are implemented. They are presented in subcestions 3.1 and 3.2, exhibit small size and independent control of the designed stopbands (ICDS). Afterwards, greater improvement in compactness is achieved by means of changing dimensions of the dual-band bandstop filter. Steps of the further miniaturization process are exposed and the impact of the alterations on the filter response is extensively investigated in subsection 3.3.
3.1. ICDS dual-band bandstop waveguide filter and equivalent electrical circuit
Specified center frequencies of the ICDS dual-band bandstop filter are f01=9 GHz and f02=11 GHz. Achieved FBWs are 5.77% and 5.27% for the lower and upper stopband, respectively. 3D models of the non-miniaturized ICDS (nmICDS) and miniaturized ICDS (mICDS) dual-band bandstop filter are presented in Figure 4. For the design of the upper stopband, length of the used QWRs is d21=2.58 mm and the distance between them is
R2=8 mm. Other dimensions are the same as for the QWRs for the lower stopband and given in subsection 2.1. Dimensions of the printed-circuit insert are ape=44.66 mm and
bpe=10.16 mm.
Figure 4.
For the nmICDS dual-band bandstop filter design, QWRs for different stopbands are attached to the same waveguide wall. The unwanted coupling between these resonators is overcome with minimal spacing of Ds = 12.5 mm. Therefore, center frequencies and bandwidths of the dual-band filter are independently tunable. Thus, the whole filter is considered as a cascade connection of the single-band bandstop filters. Total length of the filter is 0.876 λg, where λg designates the guided wavelength at the center frequency of the lower stopband.
In order to reduce the footprint of the ICDS dual-band filter, QWRs for different stopbands are attached to the opposite waveguide walls. In that manner, mICDS dual-band bandstop filter is designed. The unwanted coupling is avoided by shifting the QWRs for specified stopband along the upper waveguide wall. Herein, minimal shift is S=12 mm. Obtained frequency response is fully coinciding with the response of the nmICDS filter, but the occupying area of the filter decreased to 0.512 λg.
Equivalent circuit of the nmICDS dual-band bandstop filter is the cascade of the equivalent networks of single-band filters with the considered center frequencies, as shown in Figure 5a. The impedances of ports correspond to the wave impedance at 10 GHz, which is the mean value of the center frequencies of the lower and upper stopband. Comparison of the frequency responses for the 3D EM model and its equivalent circuit is given in Figure 5b. Equivalent circuit elements’ values of the filter at 11 GHz are given in Table 2. The values of the circuit elements of filter at 9 GHz remain unchanged (Table 1).
Figure 5.
3.2. ICDS triple-band bandstop waveguide filter
Following the proposed design procedure, a waveguide filter with three independently tunable stopbands is designed. Center frequencies are targeted at f01=9 GHz, f02=10 GHz and f03=11 GHz, while achieved bandwidths of the corresponding stopbands are FBW1=5.77 %, FBW2=5.4%, FBW3=5.27 %. 3D model of the ICDS triple-band bandstop filter is presented in
© 2016 American Geophysical Union. All rights reserved.

Confidential manuscript submitted to replace this text with name of AGU journal
Figure 6. Comparison of frequency responses for the triple-band ICDS bandstop filter and single-band filters for each specified center frequency is given in Figure 7.
For the design of the middle stopband, QWRs of length d31=2.98 mm are used, and the distance between them is R3=8 mm. Other dimensions remain unchanged and they are given in subsection 3.1. Length of the printed-circuit insert increased to ape2=54.86 mm, while bpe=10.16 mm is the same as for the previously presented inserts. Among QWRs disposed for the design of the specified stopbands, QWRs of smaller length are attached to the same waveguide wall, since such arrangement provides smaller occupied area of the filter. In the considered case of triple-band filter, QWRs with the resonating frequencies of 10 GHz and 11 GHz are attached to the lower waveguide wall, while their mutual distance is
Sd =12.9 mm. In that manner, the unwanted coupling is eliminated and designed stopbands can be independently controlled. Overall length of the triple-band ICDS bandstop filter is 0.86 λg, where λg designates the guided wavelength at the center frequency of the lowest stopband.
Figure 6.
Figure 7.
3.3 Miniaturization of the dual-band bandstop filter
Main goal is to preserve the characteristics of the ICDS dual-band bandstop filter, but to decrease the total length of the filter. The first step towards improving compactness is removal of the shift of the QWRs for specified stopband (referred to as S in mICDS filter, see Figure 4) along the waveguide wall. 3D model of the proposed ultra-compact (UC) dual-band bandstop filter is shown in Figure 8a. As seen from the figure, QWRs are positioned one below another but printed on a different sides of the insert. Hence, they influence each other’s performance and as a consequence, stopbands cannot be independently tuned. It was observed that bandwidth of the upper stopband decreased, while center frequencies and bandwidth of the lower stopband remained unchanged. Then, modifications of dimensions of the QWRs are made to reduce the length of the filter, while simultaneously maintaining the characteristics of the ICDS filter. Frequency response of the obtained UC dual-band bandstop filter is given in Figure 8b. Influence of the geometrical parameters on the response of the UC dual-band filter is given in Table 3. Dimensions of the lower stopband are: d11=2.75 mm, d12=1.3 mm, c11=0.1 mm, l=0.5 mm, m=1.08 mm, R1=8.5 mm, while the dimensions for the upper stopband are d21=2.55 mm, d12=1.3 mm, c21=0.25 mm, l=0.5 mm, p=0.45 mm and R2=7.8 mm. Total length of the filter is 0.295 λg.
Figure 8.
We investigated different possibilities for further miniaturization, which included the straight form of the QWRs and change of the substrate used for implementation of the QWRs. The filter design with QWRs in the straight form exhibits significantly wider achieved bandwidths when compared to the case when QWRs are implemented as folded elements. So, the interspace between the QWRs had to be increased in order to preserve the characteristics of the proposed filter. It has been found that the total length of the filter with QWRs in the straight form is greater than the length of our filter. Consequently, our design appears to be more convenient for miniaturization. The same effect is observed for substrates with higher permitivitty (εr). Higher εr makes the length of the printed QWRs shorter, but the
bandwidth becomes significantly wider. Therefore, we have to increase the distance between
© 2016 American Geophysical Union. All rights reserved.
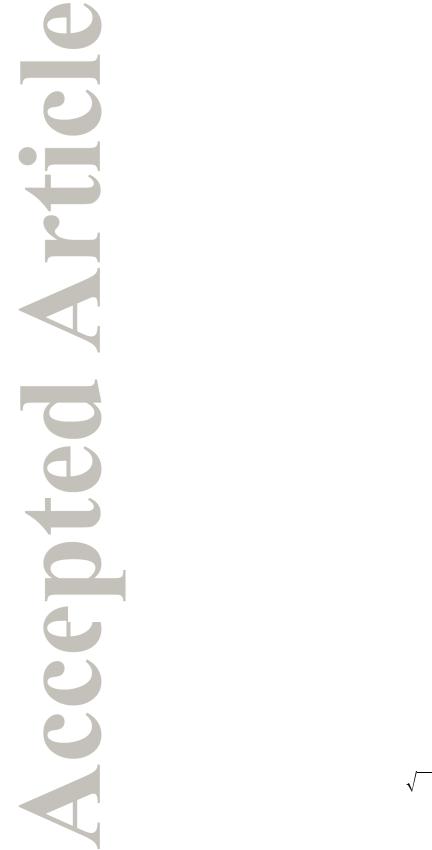
Confidential manuscript submitted to replace this text with name of AGU journal
the QWRs, which in turn increases the length of the filter. As a consequence, that filter is longer than our proposed realization.
4. Experimental Verification
In order to validate the proposed design, an E-plane mICDS dual-band bandstop waveguide filter is fabricated. The frequency response is measured with Agilent N5227A network analyzer. A photograph of the fabricated filter is given in Figure 9a, and the comparison of the simulated and measured responses is given in Figure 9b. There is a good agreement between the simulated and measured results in terms of the center frequencies of the filter and the achieved bandwidths. Slight discrepancies are observed in terms of the passband insertion loss, which occurred as a consequence of the losses within the waveguide walls and transitions from waveguide WR-90 to SMA connectors (waveguide-to-coaxial adapters). These losses haven’t been taken into account during the 3D EM analysis of the considered filter. Total length of the proposed filter is 0.512 λg. About 41% of the size reduction is achieved compared with the nmICDS filter, where QWRs for different stopbands are grounded to the same waveguide wall.
In comparison with the design examples of the E-plane dual-band waveguide filters with independently tunable stopbands, the proposed filter exhibits a smaller size than the filter of length of 3.77 λg [Lopez-Villarroya, 2012], and W-band filter of length of 0.805 λg
[Jin and Xue, 2015].
When a compact size is the main concern, the proposed UC dual-band bandstop filter is miniaturized up to 0.295 λg (Figure 8a). Significant size reduction is achieved compared to the nmICDS and mICDS dual-band filter proposed herein, about 66% and 43%, respectively. Due to the proximity of the QWRs, UC dual-band bandstop filter doesn’t exhibit independent tunability of the center frequencies and bandwidths.
Compared with the K-band dual-band bandstop filter of length of 0.328 λg presented in [Jin and Xue, 2015], where it is not possible to independently control the stopbands due to the arrangement of the used FSRRs, the UC dual-band bandstop filter proposed in this paper is smaller in size.
Figure 9.
The power-handling capability is an important design requirement for filters used in communication systems. For the proposed E-plane mICDS dual-band bandstop waveguide filter, the maximum power that filter can handle is estimated. In terms of the dielectric breakdown, it was calculated that the critical magnitude of electric field at which the breakdown of material occurs is higher than the value of the critical field for the breakdown of air. Then, the critical value of the electric field for the air breakdown Ecr=3 MV/m is considered for the power handling capability estimation. The electric field distribution inside the waveguide filter has been computed using WIPL-D software. The input power of the dominant TE10 mode is set to P0=1W and the maximal intensity of the electric field is examined for all resonators, and it was found that the maximum value of the electric field is Emax=94.78 KV/m. The maximum electrical field, in the i-th resonator of the filter, for input
power P0 can be scaled as shown in [Yu, 2007] to: Emax,i P0 . In a similar way, the electric field slightly less than the critical value Ecr can be related to the maximum value of the power
that filter can handle Ptmax. The value of Ptmax can be determined from the ratio of the mentioned electric field strengths. For our considered filter, the estimated value is Ptmax=1003
W.
© 2016 American Geophysical Union. All rights reserved.

Confidential manuscript submitted to replace this text with name of AGU journal
5. Conclusions
In this paper, a novel miniaturized E-plane ICDS multi-band bandstop waveguide filter design using QWRs has been proposed. As a proof of concept, E-plane ICDS dual-band and triple-band bandstop waveguide filters have been designed. Center frequencies can be flexibly adjusted by the length of the corresponding QWRs. As for the ICDS dual-band bandstop filter, connection of the QWRs for different stopbands to the opposite waveguide walls has resulted in about 41% of the size reduction, compared to the case where they are connected to the same waveguide wall. Designed miniaturized ICDS dual-band bandstop filter has been fabricated and measured. The filter is 0.512 λg in length, featuring a more compact size in comparison with the E-plane dual-band bandstop waveguide filters with independently tunable stopbands reported in literature.
For the triple-band ICDS filter design, independently tunable stopbands are provided by shifting the QWRs for the specified stopband along the upper waveguide wall, while QWRs for other stopbands are mutually distanced and grounded to the lower waveguide wall.
Efficient way for further miniaturization of the dual-band bandstop filter is removal of the shift of the QWRs along the upper waveguide wall. Consequently, flexible tuning of the stopbands is limited. To preserve the characteristics of the ICDS dual-band filter while reducing the occupied area, dimensions of the QWRs have been changed. Influence of the performed alterations on the filter response parameters has been thoroughly investigated. Obtained ultra-compact E-plane dual-band bandstop waveguide filter has length of 0.295 λg. It has been found that about 66% and 43% of the size reduction is achieved in comparison with the non-miniaturized and miniaturized ICDS dual-band bandstop filter. Obtained compact dual-band bandstop filter is also smaller than the E-plane dual-band bandstop waveguide filters proposed in literature.
Novel equivalent electrical circuit has been proposed for fast filter design. As for the dual-band bandstop filter, it is in the form of a cascade of the equivalent networks of the single-band filters. The equivalent circuit has been used for the fast fine tuning of the filter design and the number of time-consuming 3D EM simulations has been reduced. Proposed equivalent circuit provides highly accurate results, while grants significant savings in terms of simulation time and utilization of hardware resources.
Acknowledgments and Data
All relevant data necessary to understand, reproduce and evaluate the results reported in the article are contained within the presented manuscript, tables and figures. This work was supported by the Ministry of Education, Science and Technological Development of the Republic of Serbia under Grant TR32005.
References
Baena, J. D., J. Bonache, F. Martin, R. M. Sillero, F. Falcone, T. Lopetegi, M. A. G. Laso, J. Garcia-Garcia, I. Gil, M. F. Portillo and M. Sorolla (2005), Equivalent-circuit models for split-ring resonators and complementary split-ring resonators coupled to planar transmission lines, IEEE Trans. Microwave Theory Tech., 53(4), 1451-1461.
Cameron, R. J., C. M. Kudsia, and R. R. Mansour (2007), Microwave Filters for Communication Systems: Fundamentals, Design and Applications, Wiley, Chichester, England.
Canete Rebenaque, D., M. Martinez-Mendoza, J. L. Gomez Tornero, J. Pascual-Garcia, and A. Alvarez Melcon (2008), Analysis and implementation of different topologies of
© 2016 American Geophysical Union. All rights reserved.

Confidential manuscript submitted to replace this text with name of AGU journal
transversal filters in planar technology, Radio Sci., 43(4), RS4021, doi:10.1029/2007RS003819.
Coves, A., G. Torregrosa-Penalva, A. A. San-Blas, M. A. Sanchez-Soriano, A. Martellosio, E. Bronchalo and M. Bozzi, (2016), A novel band-pass filter based on a periodically drilled SIW structure, Radio Sci., 51(4), 328-336, doi:10.1002/2015RS005874.
Goussetis, G., R. Lopez-Villaroya, E. Doumanis, O. S. Arowolo, J.-S. Hong (2011), Quality factor of E-plane periodically loaded waveguide resonators and filter applications“, IET Microwaves, Antennas & Propagations, 5(7), 818-822.
Hong, J.-S. (2011), Microstrip filters for RF/microwave applications, John Wiley & Sons, NJ.
Jin, J. Y., X. Q. Lin, Y. Jiang, and Q. Xue (2015), A novel compact E-plane waveguide filter with multiple transmission zeros, IEEE Trans Microwave Theory Tech., 63(10), 33743380.
Jin, J. Y., and Q. Xue (2015), A Type of E-plane filter using folded split ring resonators (FSRRs), in Proc. Asia-Pacific Microwave Conference (APMC) 2015, 2, 1-3.
Jin, J.Y., X. Q. Lin and Q. Xue (2016), A Miniaturized Evanescent Mode Waveguide Filter Using RRRs, IEEE Trans. Microwave Theory Tech., 64(7), 1989-1996.
Jitha, B., C. S. Nimisha, C. K. Aanandan, P. Mohanan, and K. Vasudevan (2006), SRR loaded waveguide band rejection filter with adjustable bandwidth, Microw. Opt. Tecnol. Lett., 48(7), 1427-1429.
Lopez-Villarroya, R. (2012), E-plane parallel coupled resonators for waveguide bandpass filter applications, Ph.D. dissertation, Heriot-Watt University, Edinburgh, UK.
Lutovac, M.D., D. V. Tošić, and B. V. Evans (2001), Filter Design for Signal Processing using MATLAB and Mathematica, Upper Saddle River, NJ: Prentice Hall; Translated in Chinese. Beijing, P. R. China: Publishing House of Electronics Industry, PHEI; 2004.
Martinez Mendoza, M., D. Martinez Martinez, D. Canete Rebenaque and A. Alvarez-Melcon (2015), Enhanced topologies for the design of dual-mode filters using inductive waveguide structures, Radio Sci., 50(1), 66-77.
Mrvić, M., M. Potrebić, D. Tošić, and Z. Cvetković (2014), E-plane microwave resonator for realisation of waveguide filters, in Proceedings of XII International SAUM Conference on Systems, Automatic Control and Measurements (SAUM 2014), pp. 205–208, Niš, Serbia.
Ortiz, N., J.D. Baena, M. Beruete, F. Falcone, M.A.G. Laso, T. Lopetegi, R. Marques, F. Martin, J. Garcia-Garcia, and M. Sorolla (2005), Complementary split-ring resonator for compact waveguide filter design, Microw. Opt. Technol. Lett., 46(1), 88-92.
Pons Abenza, A., M. Martinez-Mendoza, F. D. Quesada Pereira, and A. Alvarez-Melcon (2016), Design of manifold multiplexers in all-inductive dual-mode rectangular waveguide technology using the coupling matrix formalism, Radio Sci., 51, 1065–1080, doi:10.1002/2016RS005999.
Stefanovski, S., M. Potrebić, and D. Tošić (2015), A novel design of E-plane bandstop waveguide filter using quarter-wave resonators, Optoelectron. Adv. Mat., 9(1-2), 87-93.
Stefanovski Pajović, S., M. Potrebić, and D. Tošić (2015), Microwave bandpass and bandstop waveguide filters using printed-circuit discontinuities, in Proceedings of the 23rd Telecommunications Forum (TELFOR 2015), pp. 520-527, Belgrade, Serbia.
Stefanovski, S., M. Potrebić, and D. Tošić (2014), A novel design of dual-band bandstop waveguide filter using split ring resonators, J Optoelectron. Adv. Mat., 16(3-4), 486-493.
Yu, M. (2007), Power-handling capability for RF filters, IEEE Microwave Magazine, 8(5), 88-97.
© 2016 American Geophysical Union. All rights reserved.

Confidential manuscript submitted to replace this text with name of AGU journal
Table 1. Equivalent circuit elements' values of the filter at 9 GHz
|
Parameter |
|
L (nH) |
Lm (nH) |
C (pF) |
Cm (pF) |
D1 (mm) |
De1 (mm) |
|
|
|
|
|
|
|
|
|
|
Value |
0.757 |
0.00371 |
0.4136 |
0.00038 |
12.35 |
5.255 |
|
|
|
|
|
|
|
|
|
|
© 2016 American Geophysical Union. All rights reserved.