
Книги по МРТ КТ на английском языке / Functional Neuroimaging in Child Psychiatry Ernst 1 ed 2000
.pdf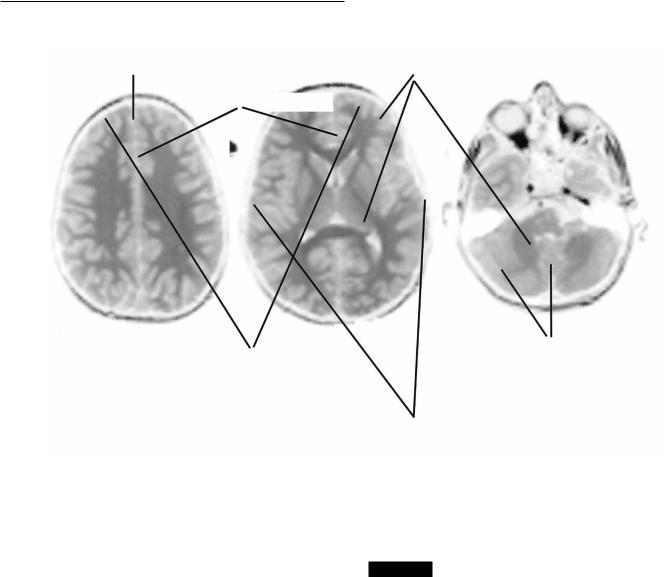
184 D. C. Chugani
[18F]-labeled DOPA |
[11C]-AMT |
Medial prefrontal |
Dentatothalamocortical pathway |
|
[18F]-FDG |
|
Anterior cingulate |
|
SPECT blood ¯ow |
SPECT blood ¯ow |
Vermis & right cerebellum |
Frontal hypoperfusion |
|
[18F]-FDG
Superior temporal hypometabolism
Fig. 10.3. Brain regions identiWed as abnormal in autistic subjects by various functional imaging modalities. Frontal, medial prefrontal, temporal, and anterior cingulate cortical regions have been implicated with the various functional tracers (AMT, '-methyltryptophan; FDG, Xuorodeoxyglucose; DOPA, dihydroxyphenylalanine). Abnormalities have also been reported in subcortical regions such as the thalamus and cerebellum. A convergence of the data from the various imaging modalities to provide a unifying hypothesis of brain mechanisms responsible for autism has not yet emerged.
puberty (Tanner et al., 1976). In addition, the higher prevalence of autism in males suggests an eVect of sex in this disorder. Likewise, maturational eVects necessitate new mathematical approaches for analyzing cross-sectional data from subjects of diVerent ages (for example see Muzik et al., 1999).
Alternatively, and perhaps ideally, longitudinal studies need to be undertaken to appreciate changes in functional brain activity with development more fully. Larger samples need to be examined. Although careful behavioral evaluation is essential to conWrm the diagnosis, twin studies showing large diVerences in phenotype between identical twins suggest it is unlikely that behavioral methods will allow further reWnement of subgroups of subjects with autism of diVering etiologies. Genetic studies, however, may lead to the identiWcation of new underlying etiologies, adding to the list of diseases associated with autism, and may aid in the subgrouping of subjects with more uniform neuroimaging abnormalities.
iReferencesi
Anderson, G. M. (1994). Studies on the neurochemistry of autism. In The Neurobiology of Autism. eds. M. L. Bauman and T. L. Kemper, pp. 227±42. Baltimore: Johns Hopkins University Press.
American Psychiatric Association (1987). Diagnostic and Statistical
Manual III-Revised. Washington, DC: American Psychiatric
Association.
American Psychiatric Association (1994). Diagnostic and Statistical
Manual IV, 4th edn. Washington, DC: American Psychiatric
Association.
Asperger, H. (1994). Die ªAutistischen Psychopathenº im Kindesalter. Arch. Psychiatr. Nervenkr., 117, 76±136.
Bailey, A., Le Couteur, A., Gottesman, I. and Bolton, P. (1995). Autism as a strongly genetic disorder: evidence from a British twin study. Psychol. Med., 25, 63±77.
Bailey, A., Phillips, W. and Rutter, M. (1996). Autism: towards an integration of clinical genetic, neuropsychological, and neurobiological perspectives. J. Child Psychol. Psychiatry, 37, 89±126.

Autism 185
Bauman, M. and Kemper, T. L. (1985). Histoanatomic observations of the brain in early infantile autism. Neurology, 35, 866±75.
Bauman, M. L. and Kemper, T. L. (1994). Neuroanatomic observations of the brain in autism. In The Neurobiology of Autism, eds. M. L. Bauman and T. L. Kemper, pp. 119±41. Baltimore: Johns Hopkins University Press.
Baxter, L. R., Mazziotta, J. C., Phelps, M. E. et al. (1987). Cerebral glucose metabolic rates in normal human females versus normal males. Psychiatr. Res., 21, 237±45.
Bennett-Clarke, C. A., Leslie, M. J., Lane, R. D. and Rhoades, R. W. (1994). EVect of serotonin depletion on vibrissae-related patterns in the rat's somatosensory cortex. J. Neurosci., 14, 7594±607.
Bennett-Clarke, C. A., Chiaia, N. L. and Rhoades, R. W. (1996). Thalamocortical aVerents in rat transiently express high-aYnity serotonin uptake sites. Brain Res., 733, 301±6.
Blue, M. E., Erzurumlu, R. S. and Jhaveri, S. (1991). A comparison of pattern formation by thalamocortical and serotonergic aVerents in the rat barrel Weld cortex. Cereb. Cortex, 1, 380±9.
Bottomley, P. A. and Hardy, C. J. (1989). Rapid, reliable in vivo assays of human phosphate metabolites by nuclear magnetic resonance. Clin. Chem., 35, 392±5.
Bourgeois, J.-P., Goldman-Rakic, P. S. and Rakic, P. (1994). Synaptogenesis in the prefrontal cortex of rhesus monkeys.
Cereb. Cortex, 4, 78±96.
Bryson, S. E. (1996). Brief report: epidemiology of autism. J. Autism Dev. Disorders, 26, 165±7.
Buchsbaum, M. S., Siegel, B. V., Wu, J. C., Hazlett, E., Sicotte, N. and Haier, R. (1992). Brief report: attention performance in autism and regional brain metabolic rate assessed by positron emission tomography. J. Autism Dev. Disorders, 22, 115±25.
Cases, O., Seif, I., Grimsby, J. et al. (1995). Aggressive behavior and altered amounts of brain serotonin and norepinephrine in mice lacking MAOA. Science, 268, 1763±6.
Cases, O., Vitalis, T., Seif, I., de Maeyer, E., Sotelo, C. and Gaspar, P. (1996). Lack of barrels in the somatosensory cortex of monoamine oxidase A-deWcient mice: role of a serotonin excess during the critical period. Neuron, 16, 297±307.
Chiron, C., Raynaud, C., Maziere, B. et al. (1992). Changes in regional cerebral blood Xow during brain maturation in children and adolescents. J. Nucl. Med., 33, 696±703.
Chiron, C., Leboyer, M., Leon, F., Jambaque, I., Nuttin, C. and Syrota, A. (1995). SPECT of the brain in childhood autism: evidence for a lack of normal hemispheric asymmetry. Dev. Med. Child Neurol., 37, 849±60.
Chugani, D. C., Muzik, O., Chakraborty, P. K., Mangner, T. J. and Chugani, H. T. (1996a). Brain serotonin synthesis measured with [11C]-methyl-tryptophan positron emission tomography in normal and autistic adults. Soc. Neurosci. Abst., 22, 22.
Chugani, D. C., Muzik, O., Rothermel, R. et al. (1997). Altered serotonin synthesis in the dentatothalamo-cortical pathway in autistic boys. Ann. Neurol., 14, 666±9.
Chugani, D. C., Muzik, O., Chakraborty, P. K., Mangner, T. and Chugani, H. T. (1998). Human brain serotonin synthesis capacity measured in vivo with alpha-[C-11]methyl-l-tryptophan.
Synapse, 28, 33±43.
Chugani, D. C., Muzik, O., Behen, M., et al. (1999a). Developmental changes in brain serotonin synthesis capacity in autistic and non-autistic children. Ann. Neurol., 45, 287±95.
Chugani, D. C., Sundram, B. S., Behen, M., Lee, M.-L. and Moore, G. J. (1999b). Evidence of altered energy metabolism in autistic children. Prog. Neuropsychopharmacol. Biol. Psychiatry, 23, 635±41.
Chugani, H. T., Phelps, M. E. and Mazziotta, J. C. (1987). Positron emission tomography study of human brain functional development. Ann. Neurol., 22, 487±97.
Chugani, H. T., da Silva, E. and Chugani, D. C. (1996b). Infantile spasms: III. Prognostic implications of bilateral hypometabolism on positron emission tomography. Ann. Neurol., 39, 643±9.
Ciaranello, A. L. and Ciaranello, R. D. (1995). The neurobiology of infantile autism. Annu. Rev. Neurosci., 18, 101±28.
Cohen, Z., Tsuiki, K., Takada, A., Beaudet, A., Diksic, M. and Hamel, E. (1995). In vivo-synthesis radioactively labeled '-methyl serotonin as a selective tracer for visualization of brain serotonin neurons. Synapse, 21, 21±8.
Cook, E. H., Rowlett, R., Jaselskis, C. and Leventhal, B. L. (1992). Fluoxetine treatment of children and adults with autistic disorder and mental retardation. J. Am. Acad. Child Adolesc. Psychiatry, 31, 739±45.
Cook, E. H., Courchesne, R., Lord, C. et al. (1997). Evidence of linkage between the serotonin transporter and autistic disorder.
Mol. Psychiatry, 2, 247±50.
Courchesne, E., Courchesne, R. Y., Press, G. A., Hesselink, J. R. and Jernigan, T. L. (1988). Hypoplasia of cerebellar vermal lobules VI and VII in autism. N. Engl. J. Med., 813, 1349±54.
de Volder, A., Bol, A., Michel, C., Congneau, M. and GoYnet, A. (1987). Brain glucose metabolism in children with the autistic syndrome: positron tomography analysis. Brain Dev., 9, 581±7.
Diksic, M., Nagahiro, S., Sourkes, T. L. and Yamamoto, Y. L. (1990). A new method to measure brain serotonin synthesis in vivo. I. Theory and basic data for a biological model. J. Cereb. Blood Flow Metab., 9, 1±12.
DiLavore, P. C., Lord, C. and Rutter, M. (1995). The pre-linguistic autism diagnostic observation schedule. J. Autism Dev. Disorders, 25, 355±79.
EgelhoV, J. C., Ball, W. S. Jr, Koch, B. L. and Parks, T. D. (1997). Safety and eYcacy of sedation in children using a structured sedation program. Am. J. Roentgenol., 168, 1259±62.
Ernst, M., Zametkin, A., Matochik, J., Pascualvaca, D. and Cohen, R. (1997). Low medial prefrontal dopaminergic activity in autistic children. Lancet, 350, 638.
Ernst, M., Freed, M. E. and Zametkin, A. J. (1998). Health hazards of radiation exposure in the context of brain imaging research: special consideration for children. J. Nucl. Med., 39, 689±98.
Folstein, S. E. and Piven, J. (1991). Etiology of autism: genetic inXuences. Pediatrics, 87, 767±73.
Folstein, S. E. and Rutter, M. L. (1977). Infantile autism: a genetic study of 21 twin pairs. J. Child Psychol. Psychiatry, 18, 297±321.
Folstein, S. E. and Rutter, M. L. (1988). Autism: familial aggregration and genetic implications. J. Autism Dev. Disorders, 18, 3±30.
Friedman, E. (1969). The ªautistic syndromeº and phenylketonuria. Schizophrenia, 1, 249±61.

186 D. C. Chugani
George, M., Costa, D., Kouris, K., Ring, H. and Ell, P. (1992). Cerebral blood Xow abnormalities in adults with infantile autism. J. Nerv. Mental Dis., 180, 413±17.
Gillberg, C. (1989). Asperger syndrome in 23 Swedish children. Dev. Med. Child Neurol., 31, 520±31.
Goldman-Rakic, P. S. and Brown, R. M. (1982). Postnatal development of monoamine content and synthesis in the cerebral cortex of rhesus monkeys. Dev. Brain Res., 4, 339±49.
Gordon, C. T., Rapoport, J. L., Hamburger, S. D., State, R. C. and Mannheim, G. B. (1992). DiVerential response of seven subjects with autistic disorder to clomipramine and desipramine. Am. J. Psychiatry, 149, 363±6.
Gordon, C. T., State, R. C., Nelson, J. E., Hamburger, S. D. and Rapoport, J. L. (1993). A double-blind comparison of clomipramine, desipramine and placebo in the treatment of autistic disorder. Arch. Gen. Psychiatry, 50, 441±7.
Hagberg, B. (1985). Retts syndrome: prevalence and impact on progressive severe mental retardation in girls. Acta Paediatr. Scand.,
74, 405±8.
Happé, F., Ehlers, S., Fletcher, P. et al. (1996). ªTheory of mindº in the brain. Evidence from a PET scan study of Asperger syndrome. Neuroreport, 8, 197±201.
Hashimoto, T., Tayama, M., Miyazaki, M. et al. (1997). DiVerences in brain metabolites between patients with autism and mental retardation as detected by in vivo localized proton magnetic resonance spectroscopy. J. Child Neurol., 12, 91±6.
Haznedar, M., Buchsbaum, M., Metzger, M., Solimando, A., Spiegel-Cohen, J. and Hollander, E. (1997). Anterior cingulate gyrus volume and glucose metabolism in autistic disorder. Am. J. Psychiatry, 154, 1047±50.
Heh, C. W. C., Smith, R., Wu, J. et al. (1989). Positron emission tomography of the cerebellum in autism. Am. J. Psychiatry, 146, 242±5.
Herold, S., Frackowiak, R., Le Couteur, A., Rutter, M. and Howlin, P. (1988). Cerebral blood Xow and metabolism of oxygen and glucose in young autistic adults. Psychol. Med., 18, 823±31.
HoVman, E. J., Huang, S. C. and Phelps, M. E. (1979). Quantitation in positron emission computed tomography: 1. EVect of object size. J. Comput. Assist. Tomogr., 3, 299±308.
Horwitz, B., Rumsey, J., Grady, C. and Rapoport, S. (1988). The cerebral metabolic landscape in autism. Intercorrelations of regional glucose utilization. Arch. Neurol., 45, 749±55.
Huttenlocher, P. R. (1979). Synaptic density in human frontal cortex
± developmental changes and eVects of aging. Brain Res., 163, 195±205.
Huttenlocher, P. R. (1990). Morphometric study of human cerebral cortex development. Neuropsychologia, 28, 517±27.
Huttenlocher, P. R. and de Courten, C. (1987). The development of synapses in the striate cortex of man. Hum. Neurobiol., 6, 1±9.
Huttenlocher, P. R., de Courten, C., Garey, L. J. and van der Loos, H. (1982). Synaptogenesis in human visual cortex: evidence for synapse elimination during normal development. Neurosci. Lett., 33, 247±52.
IRCP (1988). Radiation dose to patients from radiopharmaceutical.
Annals of the International Commission on Radiological Protection, Publication 53, p. 15, New York: Pergamon Press.
Jacobs, B., Chugani, H. T., Allada, V. et al. (1995). Developmental changes in brain metabolism in sedated rhesus macaques and vervet monkeys revealed by positron emission tomography.
Cereb. Cortex, 5, 222±33.
Jenkins, B., Koroshetz,W., Beal, M. F. and Rosen, B. (1993). Evidence for an energy metabolism defect in Huntington's disease using localized proton spectroscopy. Neurology, 43, 2685±95.
Kanner, L. (1943). Autistic disturbances of aVective contact.
Nervous Child, 2, 217±50.
Killiany, R. J. and Moss, M. B. (1994). Memory function and autism. In The Neurobiology of Autism, eds. M. L. Bauman and T. L. Bauman, pp. 170±94. Baltimore: Johns Hopkins Press.
Landa, R., Piven, J., Wzorek, M. M. et al. (1990). Social language use in parents of autistic individuals. Psychol. Med., 22, 245±54.
Landa, R., Folstein, S. and Issacs, C. (1991). Spontaneous narrativediscourse performance of parents of autistic individuals. J. Speech Hearing Res., 34, 1339±45.
Landa, R., Piven, J., Wzorek, M. M., Gayle, J. O., Chase, G. A. and Folstein, S. E. (1992). Social language use in parents of autistic individuals. Psychol. Med., 22, 1245±54.
Lauder, J. M. and Krebs, H. (1978). Serotonin as a diVerentiation signal in early embryogenesis. Dev. Neurosci., 1, 15±30.
Lebrand, C., Cases, O., Adelbrecht, C. et al. (1996). Transient uptake and storage of serotonin in developing thalamic neurons.
Neuron, 17, 823±35.
Le Couteur, A., Bailey, A., Goode, S. et al. (1996). A broader phenotype of autism: the clinical spectrum in twins. J. Child Psychol. Psychiatr. Allied Disciplines, 37, 785±801.
Lidow, M. S., Goldman-Rakic, P. S. and Rakic, P. (1991). Synchronized overproduction of neurotransmitter receptors in diverse regions of the primate cerebral cortex. Proc. Natl. Acad. Sci. USA, 88, 10218±21.
Lord, C. (1995). Follow-up of two-year-olds referred for possible autism. J. Child Psychol. Psychiatr. Allied Disciplines, 36, 1365±82.
Lord, C. and Schopler, E. (1989). The role of age at assessment, developmental level, and test in the stability of intelligence scores in young autistic children. J. Autism Dev. Disorders, 19, 483±99.
Lord, C., Rutter, M., Goode, S. et al. (1989). Autism diagnostic observation schedule: a standardized observation of communicative and social behavior. J. Autism Dev. Disorders, 19, 185±212.
Lord, C., Rutter, M. and LeCouteur, A. (1994). Autism diagnostic interview ± revised: a revised version of diagnostic interview for caregivers of individuals with possible pervasive developmental disorders. J. Autism Dev. Disorders, 24, 659±85.
Lord, C., Pickles, A., McLennan, J. et al. (1997). Diagnosing autism: analyses of data from the autism diagnostic interview. J. Autism Dev. Disorders, 27, 501±17.
Lotspeich, L. J. and Ciaranello, R. D. (1993). The neurobiology and genetics of infantile autism. In International Review of Neurobiology, ed. R. Bradley, pp. 87±129. San Diego, CA: Academic Press.
Madras, B. K. and Sourkes, T. L. (1965). Metabolism of '-methyl- tryptophan. Biochem. Pharmacol., 14, 1499±506.
McDougle, C. J., Price, L. H., Volkmar, F. R. et al. (1992). Clomipramine in autism: preliminary evidence of eYcacy. J. Am. Acad. Child Adolesc. Psychiatry, 31, 746±50.

Autism 187
McDougle, C. J., Naylor, S. T., Cohen, D. J., Aghajanian, G. K., Heninger, G. R. and Price, L. H. (1996a). EVects of tryptophan depletion in drug-free adults with autistic disorder. Arch. Gen. Psychiatry, 53, 993±1000.
McDougle, C. J., Naylor, S. T., Cohen, D. J., Volkmar, F. R., Heninger, G. R. and Price, L. H. (1996b). A double-blind, placebo-con- trolled study of Xuvoxamine in adults with autistic disorder.
Arch. Gen. Psychiatry, 53, 1001±8.
McKelvey, J., Lambert, R., Mottron, L. and Shevell, M. (1995). Righthemisphere dysfunction in Asperger's syndrome. J. Child Neurol., 10, 310±14.
Merola, C., Albarracin, C., Lebowitz, P., Bienkowski, R. S. and Barst, S. M. (1995). An audit of adverse events in children sedated with chloral hydrate or propofol during imaging studies. Paediatr. Anaesth., 5, 375±8.
Minshew, N., Goldstein, G., Dombrowski, S., Panchalingam, K. and Pettegrew, J. (1993). A preliminary 31P MRS study of autism: evidence for undersynthesis and increased degradation of brain membranes. Biol. Psychiatry, 33, 762±73.
Minshew, N. J. Goldstein, G. and Siegel, D. J. (1997). Neuropsychologic functioning in autism: proWle of a complex information processing disorder. J. Int. Neuropsychol. Soc., 3, 303±16.
Miquel, M. C., Kia, H. K., Boni, C. et al. (1994). Postnatal development and localization of 5-HT1A receptor mRNA in rat forebrain and cerebellum. Dev. Brain Res., 80, 149±57.
Missala, K. and Sourkes, T. L. (1988). Functional cerebral activity of an analogue of serotonin formed in situ. Neurochem. Int., 12, 209±14.
Moore, G. J. (1998). Proton magnetic resonance spectroscopy in pediatric neuroradiology. Pediatr. Radiol., 11, 805±14.
Mountz, J., Tolbert, L., Lill, D., Katholi, C. and Liu, H. (1995). Functional deWcits in autistic disorder: characterization by tech- netium-99m-HMPAO and SPECT. J. Nucl. Med., 36, 1156±62.
Müller, R. A., Chugani, D. C., Behen, M. E. et al. (1998). Impairment of dentato-thalamo-cortical pathway in autistic men: language activation data from positron emission tomography. Neurosci. Lett., 245, 1±4.
Müller, R. A., Behen, M. E., Rothermel, R. D. et al. (1999). Brain mapping of language and auditory perception in high-function- ing autistic adults: a PET study. J. Autism Dev. Disorders, 29, 19±31.
Muzik, O., Chugani, D. C., Chakraborty, P. K., Mangner, T. and Chugani, H. T. (1997). Analysis of [C-11]alpha-methyl-trypto- phan kinetics for the estimation of serotonin synthesis rate in vivo. J. Cereb. Blood Flow Metab., 17, 659±69.
Muzik, O., Ager, J., Janisse, J., Shen, C., Chugani, D. C. and Chugani, H. T. (1999). A mathematical model for the analysis of cross-sec- tional brain glucose metabolism data in children. Prog. NeuroPsychopharmacol. Biol. Psychiatry, 23, 589±600.
Nishizawa, S., Benkelfat, C., Young, S. N. et al. (1997). DiVerences between males and females in rates of serotonin synthesis in human brain. Proc. Natl. Acad. Sci. USA, 94, 5308±13.
Osterheld-Haas, M. C. and Hornung, J. P. (1996). Laminar development of the mouse barrel cortex: eVects of neurotoxins against monoamines. Exp. Brain Res., 110, 183±95.
Ozbayrak, K. R., Kapucu, O., Erdem, E. and Aras, T. (1991). Left occipital hypoperfusion in a case with Asperger syndrome. Brain Dev., 13, 454±6.
Payton, J. B., Steele, M. W., Wenger, S. L. and Minshew, N. J. (1989). The fragile X marker and autism in perspective. J. Am. Acad. Child Adolesc. Psychiatry, 28, 417±21.
Piven, J. and Folstein, S. (1994). The genetics of autism. In The Neurobiology of Autism, eds. M. L. Bauman and T. L. Kemper, pp. 18±44. Baltimore: Johns Hopkins University Press.
Piven, J., Gayle, J., Landa, R.,Wzorek, M. and Folstein, S. (1991). The prevalence of fragile X in a sample of autistic individuals diagnosed using a standardized interview. J. Am. Acad. Child Adolesc. Psychiatry, 30, 825±30.
Piven, J., Wzorek, M., Landa, R. et al. (1994). Personality characteristics of the parents of autistic individuals. Psychol. Med., 24, 783±95.
Piven, J., Palmer, P., Jacobi, D., Childress, D. and Arndt, S. (1997). Broader autism phenotype: evidence from a family history of multiple-incidence autism families. Am. J. Psychiatry, 154, 185±90.
Rakic, P., Bourgeois, J.-P., EckenhoV, M. F., Zecevic, N. and Goldman-Rakic, P. S. (1986). Concurrent overproduction of synapses in diverse regions of the primate cerebral cortex. Science,
232, 232±5.
Reiss, A. L., Lee, J. and Freund, L. (1994). Neuroanatomy of fragile X syndrome: the temporal lobe. Neurology, 44, 1317±24.
Riikonen, R. and Amnell, G. (1981). Psychiatric disorders in children with earlier infantile spasms. Dev. Med. Child Neurol., 23, 747±60.
Ritvo, E. R., Freeman, B. J., Scheibel, A. B. et al. (1986). Lower Purkinje cell counts in the cerebella of four autistic subjects: initial Wndings of the UCLA±NSAC autopsy research report. Am. J. Psychiatry, 143, 862±6.
Rosenberg, D. R., Sweeney, J. A., Gillen, J. S. et al. (1997). Magnetic resonance imaging of children without sedation: preparation with simulation. J. Am. Acad. Child Adolesc. Psychiatry, 36, 853±9.
Rumsey, J., Duara, R., Grady, C. et al. (1985). Brain metabolism in autism. Resting cerebral glucose utilization rates as measured with positron emission tomography. Arch. Gen. Psychiatry, 42, 448±55.
Sanchez, L. E., Campbell, M., Small, A. M., Cueva, J. E., Armenteros, J. L. and Adams, P. B. (1996). A pilot study of clomipramine in young autistic children. J. Am. Acad. Child Adolesc. Psychiatry,
35, 537±44.
Santangelo, S. L. and Folstein, S. E. (1995). Social deWcits in the families of autistic probands. Am. J. Hum. Genetics, 53, 855.
Schain, R. J. and Freedman, D. X. (1961). Studies on 5-hydoxyindole metabolism in autism and other mentally retarded children. J. Pediatr., 59, 315±20.
Schifter, T., HoVman, J., Hatten, H., Hanson, M., Coleman, R. and de Long, G. (1994). Neuroimaging in infantile autism. J. Child Neurol., 9, 155±61.
Schopler, E., Reichler, R. J., de Vellis, R. F. and Daly, K. (1980). Toward objective classiWcation of childhood autism: childhood autism rating scale (CARS). J. Autism Dev. Disorders, 10, 91±103.
Sevin, J. A., Matson, J. L., Coe, D., Love, S. R., Matese, M. J. and

188 D. C. Chugani
Benavidez, D. A. (1995). Empirically derived subtypes of pervasive developmental disorders: a cluster analytic study. J. Autism Dev. Disorders, 25, 561±78.
Sherman, M., Nass, R. and Shapiro, T. (1984). Regional cerebral blood Xow in infantile autism. J. Autism Dev. Disorders, 4, 438±40.
Siegel, B. V. Jr, Asarnow, R., Tanguay, P. et al. (1992). Regional cerebral glucose metabolism and attention in adults with a history of childhood autism. J. Neuropsychiatry Clin. Neurosci., 4, 406±14.
Siegel, B., Nuechterlein, K., Abel, L., Wu, J. and Buchsbaum, M. (1995). Glucose metabolic correlates of continuous performance test performance in adults with a history of infantile autism, schizophrenics, and controls. Schizophr. Res., 17, 85±94.
Slifer, K., Cataldo, M. F., Cataldo, M. D., Llorente, A. and Gerson, A. (1993). Behavior analysis of motion control for pediatric neuroimaging. J. Appl. Behav. Anal., 26, 469±70.
Slifer, K., Bucholts, J. and Cataldo, M. D. (1994). Behavioral training of motion control on young children undergoing radiation treatment without sedation. J. Pediatr. Oncol. Nursing, 11, 55±63.
Smalley, S. L., Tanguay, P. E., Smith, M. and Gutierrez, G. (1992). Autism and tuberous sclerosis. J. Autism Dev. Disorders, 22, 339±55.
SteVenburg, S., Gillberg, C., Hellgren, L. et al. (1989). A twin study of autism in Denmark, Finland, Iceland, Norway and Sweden. J. Child Psychol. Psychiatr. Allied Disciplines, 30, 405±16.
Tanner, J. M., Whitehouse, R. H., Marubini, E. and Resele, L. F. (1976). The adolescent growth spurt of boys and girls of the Harpenden growth. Ann. Hum. Biol., 3, 109±26.
Theodore, W. H., di Chiro, G., Margolin, R., Fishbein, D., Porter, R.
J. and Brooks, R. A. (1986). Barbiturates reduce human cerebral glucose metabolism. Neurology, 36, 60±4.
Tuchman, R. F. and Rapin, I. (1997). Regression in pervasive developmental disorders: seizures and epileptiform EEG correlates.
Pediatrics, 99, 560±6.
Volkmar, F. R., Sparrow, S. S., Goudreau, D., Cicchetti, D. V., Paul, R. and Cohen, D. J. (1987). Social deWcits in autism: an operational approach using the Vineland adaptive behavior scales. J. Am. Acad. Child Adolesc. Psychiatry, 26, 156±61.
Volkmar, F. R., Klin, A., Schultz, R. et al. (1996). Asperger's syndrome. J. Am. Acad. Child Adolesc. Psychiatry, 35, 118±23.
Wing, L. (1981). Asperger's syndrome: a clinical account. Psychol. Med., 11, 115±29.
Wing, L. (1993). The deWnition and prevalence of autism. A review.
Eur. Child Adolesc. Psychiatry, 2, 61±74.
Wing, L. and Gould, J. (1979). Severe impairments of social interaction and associated abnormalities in children: epidemiology and classiWcation. J. Autism Dev. Disorders, 9, 11±29.
World Health Organization (1987). International ClassiWcation of Diseases, 10th Draft. New York: WHO.
Yan, W., Wilson, C. C. and Haring, J. H. (1997). EVects of neonatal serotonin depletion on the development of rat dentate granule cells. Dev. Brain Res., 98, 177±84.
Zilbovicius, M., Garreau, B., Tzourio, N. et al. (1992). Regional cerebral blood Xow in childhood autism: a SPECT study. Am. J. Psychiatry, 149, 924±30.
Zilbovicius, M., Garreau, B., Samson, Y. et al. (1995). Delayed maturation of the frontal cortex in childhood autism. Am. J. Psychiatry, 152, 248±52.

11
Functional imaging in childhoodonsent schizophrenia
Leslie K. Jacobsen and Alessandro Bertolino
Introduction
This chapter will begin with a review of developmental theories of schizophrenia to provide a foundation for a discussion of functional brain imaging studies of childhoodonset schizophrenia. Two examples of such studies conducted with the National Institute of Mental Health (NIMH) childhood-onset schizophrenia sample are provided, followed by a discussion of future directions. Schizophrenia with onset during late adolescence or early adulthood is referred to as ªadult-onset schizophreniaº whereas childhood-onset schizophrenia is usually deWned as onset by the age of 12 years.
Etiology of schizophrenia
Since the 1970s, etiologic hypotheses about schizophrenia have shifted away from a view of the disorder as arising from pathologic processes occurring shortly prior to the onset of symptoms leading to diagnosis toward a neurodevelopmental conceptualization of the disorder. The neurodevelopmental hypothesis of schizophrenia asserts that schizophrenia results from an interaction between normal brain maturational events and a congenital brain lesion(s) (Weinberger, 1987). This shift in thinking is the result of cumulative evidence supporting the existence of brain morphologic and functional abnormalities in schizophrenia, together with the observation that disease onset typically occurs during late adolescence or early adulthood. Neurodevelopmental hypotheses argue that some brain abnormalities in people destined to develop schizophrenia may exist from birth, although symptoms of schizophrenia do not appear until aVected brain areas and related circuitry mature (Murray and Lewis, 1987; Weinberger, 1987, 1995; Done et al., 1994; Jones et al., 1994; Murray, 1994).
One line of compelling evidence in support of the neurodevelopmental hypothesis of schizophrenia has come from animal studies. A large body of work in nonhuman primates by Patricia Goldman-Rakic and others has implicated the dorsolateral prefrontal cortex in working memory, a core aspect of cognition that is impaired in schizophrenia. In working memory tasks, information is presented and then must be recalled (Fuster and Alexander, 1971). A common paradigm used to test working memory in animals is the delayed response paradigm, wherein the animal is presented information (e.g., the location of a food pellet) that must be recalled after a delay interval. In humans, working memory is thought to provide a foundation for important higher cognitive functions, such as comprehension, thinking, and planning (Goldman-Rakic, 1994). Patients with schizophrenia perform poorly on tests of working memory, and this impairment may underlie a number of core symptoms of schizophrenia, including disorganization of thinking and behavior, an inability to modify strategies based upon experience, lack of initiative, and poverty of speech (Goldman-Rakic, 1991).
Adult monkeys with lesions of the dorsolateral prefrontal cortex perform at chance levels during tests of working memory. The perseverative errors that these animals frequently make, together with their distractibility and impulsivity, has led investigators to view them as a model for some dimensions of schizophrenia (GoldmanRakic, 1991). In keeping with predictions from neurodevelopmental hypotheses, monkeys with dorsolateral prefrontal lesions created in infancy do not show deWcits in performance on any task or in their behavior until 2 years of age (adolescence), at which point impairment appears and worsens with increasing age (Goldman, 1974). In contrast, lesions created in adolescent or adult monkeys are associated with immediate impairment in working
189
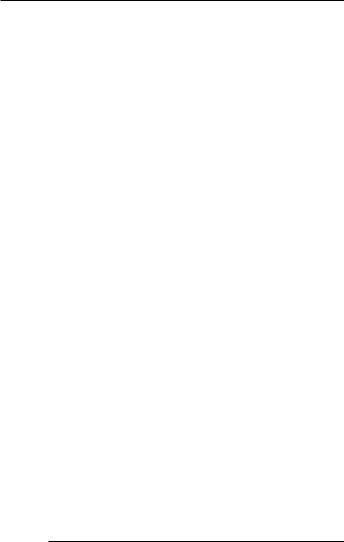
190L. K. Jacobsen and A. Bertolino
memory performance. The delayed emergence of impairment in monkeys with lesions created in infancy is thought to reXect the late maturation of the dorsolateral prefrontal cortex and, consequently, the delayed integration of this cortical region into neural circuits subserving memory, particularly mesiotemporal±prefrontal cortical circuitry. In humans, the prefrontal brain area is the last to begin myelination and continues to myelinate throughout life (Yakovlev and Le Cours, 1964). Prior to maturation of this region, delayed response performance is mediated by other brain regions; consequently deWcits related to dorsolateral prefrontal cortex lesions become apparent only once this region is mature and normally becomes more essential for working memory performance (Goldman, 1974; Goldman and Alexander, 1977).
Another animal model has been elaborated in rats (Lipska and Weinberger, 1995) in which neonatal hippocampal lesions are thought to interfere with the development of prefrontal cortex and with its integration into neural circuits. Such lesions produce a spectrum of changes in prefrontal function when the rats reach adulthood, resulting in abnormalities of cognition (Lipska et al., 1996), social behavior (Sams-Dodd et al., 1997), and gene expression (Lillrank et al., 1996). These animals become hyperresponsive to stress and to amphetamine challenge after puberty. Of particular relevance to childhood-onset schizophrenia is the observation that in certain strains of rats effects of hippocampal lesions emerge at an earlier age.
Adult-onset schizophrenia
Structural neuroimaging studies conducted in patients with onset of schizophrenia during late adolescence or early adulthood have produced a wealth of evidence in support of neurodevelopmental hypotheses of schizophrenia. The animal models described above predict that humans with schizophrenia will have smaller brain structures, particularly in frontal and temporal lobe regions, as the result of a neurodevelopmental lesion leading to failure of tissue development or to overly exuberant synaptic pruning during adolescence and/or early adulthood (Feinberg, 1982).
Consistent with this prediction, both autopsy studies and structural magnetic resonance imaging (MRI) studies in adult schizophrenia have demonstrated reduced medial temporal lobe volume, reduced frontal lobe volume, and enlarged lateral ventricles, as well as reduced midsagittal thalamic area and enlarged third ventricles (Andreasen et al., 1986, 1994a,b; Falkai and Bogerts, 1986; Jakob and Beckmann, 1989; Jeste and Lohr, 1989; Altshuler et al.,
1990; Bogerts et al., 1990a,b, 1993; Suddath et al., 1990; Arnold et al., 1991; Jernigan et al., 1991; Breier et al., 1992; Marsh et al., 1994; Zipursky et al., 1994; Flaum et al., 1995). Previous observations of basal ganglia enlargement now appear to reXect an eVect of typical neuroleptic medications, as patients subsequently switched to atypical medication show normalization of basal ganglia volume (Chakos et al., 1995). Reduced total cerebral volume has also been observed, with recent autopsy work suggesting that this reduction is largely a result of decreased neuropil throughout the cortex, reXecting decreased synaptic density (Selemon et al., 1995). Consistent with this, in vivo brain MRI studies have found widespread reductions in cortical gray, but not white, matter, which are most prominent in frontal and temporal regions (Lim et al., 1996; Sullivan et al., 1998). Furthermore, the same pattern of gray matter reduction with white matter sparing has been observed in a sample of patients with congential rubella and schizophrenia-like symptoms, suggesting that this pattern of brain dysmorphology can result from a viral disturbance of early neurodevelopment (Lim et al., 1995).
Functional neuroimaging studies
Resting-state studies of cerebral glucose metabolism and blood Xow in patients with adult-onset schizophrenia have yielded inconsistent Wndings (Chua and McKenna, 1995). Some have demonstrated reduced anterior-to-posterior ratios of metabolism in schizophrenia (Buchsbaum et al., 1984; DeLisi, et al., 1985); others have shown no group diVerences in frontal metabolism or blood Xow (Wiesel et al., 1987; Ebmeier et al., 1995), and still others have shown increased frontal metabolism in schizophrenia (Szechtman et al., 1988).
Studies measuring glucose metabolism or blood Xow during task performance have yielded more consistent Wndings. Hypofrontality has been demonstrated while schizophrenic adults performed the Wisconsin Card Sorting Test (Berman et al., 1992; Volz et al., 1997), the Tower of London (Andreasen et al., 1992), and the Continuous Performance Test (CPT) (Cohen et al., 1987; Siegel et al., 1993). SpeciWc dysfunction of prefrontallimbic neurocircuitry has been implicated in a study of monozygotic twins discordant for schizophrenia, which demonstrated that the schizophrenic twin's failure to activate prefrontal cortex during a working memory task was closely related to a reduction in left hippocampal volume (Weinberger et al., 1992). Hypofrontality has been particularly associated with deWcit symptoms, which, along with poor performance on measures of frontal lobe functioning, is thought to result from the schizophrenic
Functional imaging in childhood-onset schizophrenia |
191 |
|
|
|
|
patients' inability to activate frontal regions (Andreasen et al., 1992; Siegel et al., 1993).
Proton magnetic resonance spectroscopy (1H MRS) represents another in vivo imaging methodology that has been utilized to test the neurodevelopmental hypothesis of schizophrenia. This method permits in vivo measurement of some aspects of brain biochemistry. It detects signals arising from N-acetyl-containing moieties, mainly N- acetylaspartate (NAA). This compound is located within neurons (Urenjak et al., 1993), increases during development (Toft et al., 1994), and has been shown to be an intraneuronal marker sensitive to pharmacologic inhibition of mitochondrial energy metabolism and to a number of pathologic processes aVecting the integrity of neurons (Arnold et al., 1990; de Stefano et al., 1995; Hugg et al., 1996, Cheng et al., 1997). Since neuronal number does not signiWcantly increase after birth in humans or in monkeys, postnatal increases in NAA appear to reXect maturational processes. Proton MRS also detects signals arising from choline-containing compounds (Cho), such as glycerophosphocholine and phosphocholine (Miller, 1991; Barker et al., 1994), and from creatine plus phosphocreatine (Cre). Variation in the signal of Cho is associated with alterations in membrane turnover. Creatine and phosphocreatine buVer the ATP/ADP ratio. Previous single-voxel 1H MRS studies of patients with adult-onset schizophrenia have reported reductions of NAA signal intensity or concentration in the mesial temporal lobes (Renshaw et al., 1995; Yurgelun-Todd et al., 1996), in the region of the hippocampus (Nasrallah et al., 1994; Maier et al., 1995), and in the frontal lobe (Buckley et al., 1994), but not without debate (Buckley et al., 1994; Fukuzako et al., 1995; Stanley et al., 1996; Bartha et al., 1997).
The use of 1H MRS has evolved to permit imaging that maps signals acquired from a large number of small singlevolume elements (Duyn et al., 1993). Using this technique, reductions in NAA levels in the hippocampal area and in the dorsolateral prefrontal cortex have been demonstrated in patients with adult-onset schizophrenia (Bertolino et al., 1996, 1998a,b; Deicken et al., 1997). These Wndings were independent of drug treatment and were consistent with developmental neuronal pathology (Bertolino et al., 1996, 1997, 1998a,b).
Proton MRS imaging has also been applied to an animal model of schizophrenia to test more directly the neurodevelopmental hypothesis of this disorder (Bertolino et al., 1997). In parallel to the rat model of schizophrenia of Lipska and colleagues (1993) described above, the mesial temporal lobe was ablated bilaterally in six monkeys within 3 weeks of birth and in six monkeys at 5 years of age (adult). Subsequent 1H MRS imaging demonstrated
reduced NAA in the prefrontal cortex of animals who had undergone mesial temporal lobe ablation during infancy but not in animals undergoing this procedure as adults. These Wndings suggest that normal prefrontal cortical maturation is dependent upon neurons in this region receiving target feedback from mesiotemporal lobe neurons. This model also demonstrates that maldevelopment of prefrontal cortex and mesiotemporal±prefrontal cortical circuitry in schizophrenia could arise from early postnatal lesions of the mesiotemporal lobe (Bertolino et al., 1997).
Neuroreceptor imaging
Neurochemical brain imaging methodologies have permitted testing of the dopamine hypothesis of schizophrenia (Creese et al., 1976; Meltzer and Stahl, 1976). This hypothesis stemmed from the early observation that dopamimetic drugs, when taken in large quantities, can produce a schizo- phrenic-like psychosis. In addition, the antipsychotic potency of many neuroleptic drugs is correlated with the degree to which they bind to striatal dopamine receptors. More recent support for the involvement of dopamine in schizophrenia has come from observations of deterioration in performance on frontal lobe tasks (e.g., working memory tasks) in animals (Brozowski et al., 1979) and in humans (Stern and Langston, 1985) in whom dopaminergic aVerents to frontal lobe have been lesioned. In a recent single photon emission computed tomography (SPECT) study using the dopamine D2 receptor radioligand [123I]-iodobenzamide ([123I]-IBZM), patients with schizophrenia were found to have greater amphetamine-stimulated dopamine release than healthy controls, as measured by [123I]-IBZM displacement, indicating increased dopamine transmission in schizophrenia (Laruelle et al., 1996; Abi-Dargham et al., 1998). More recently, the eYcacy of atypical antipsychotic drugs, which have signiWcant aYnity for a wide range of receptor systems, has drawn attention to the role of other neurotransmitter systems in schizophrenia. These include serotonin (Abi-Dargham et al., 1997) and the gammaaminobutyric acid (GABA) receptor complex (Benes et al., 1997).
While considerable evidence has accumulated in support of the neurodevelopmental hypothesis of schizophrenia, this hypothesis has its limitations (Weinberger, 1987). A major challenge to any neurobiologic hypothesis of schizophrenia is to account for atypical presentations of the disorder. Such cases test the boundaries of hypotheses based upon data collected from patients with more common disease manifestations and can result in a deeper understanding of disease mechanisms.

192L. K. Jacobsen and A. Bertolino
Childhood-onset schizophrenia
Phenomenology and neurobiology
The onset of schizophrenia in childhood, usually deWned as onset by age 12, is extremely rare (Remschmidt et al., 1994) and therefore qualiWes as an atypical presentation of schizophrenia. While no large-scale epidemiologic studies using rigorous diagnostic criteria have been conducted, it has been estimated that the prevalence of childhood-onset schizophrenia may be 50 times less than that of adultonset schizophrenia (Karno and Norquist, 1989).
Because of the extreme rarity of childhood-onset schizophrenia, few systematic studies of phenomenology or neurobiology of this disorder have been conducted. Studies of phenomenology have demonstrated that, although it is rare, childhood-onset schizophrenia does occur and can be diagnosed using the same standard criteria used to diagnose adults with schizophrenia (American Psychiatric Association, 1994; Russell, 1994). Like other early-onset forms of multifactorial diseases (e.g. juvenile-onset diabetes mellitus, juvenile rheumatoid arthritis), childhood-onset schizophrenia is associated with greater disease severity and chronicity than adultonset schizophrenia (Gordon et al., 1994).
Clinical studies have demonstrated prominent premorbid developmental delays in childhood-onset schizophrenia, especially in the areas of speech and language (Alaghband-Rad et al., 1995; Asarnow et al., 1995; Hollis, 1995). In addition, transient symptoms of pervasive developmental disorder, such as hand Xapping and echolalia, are common in the prepsychotic period (Alaghband-Rad et al., 1995; Watkins et al., 1988; Russell et al., 1989). This is one area of phenomenologic distinction between childhoodand adult-onset schizophrenia, as symptoms of pervasive developmental disorder have not been reported in studies of the premorbid history of the latter (Done et al., 1994; Jones et al., 1994). The conspicuous prepsychotic developmental abnormalities seen in childhood-onset schizophrenia are consistent with a neurodevelopmental etiology, with the pattern suggesting that language-related brain areas, such as those in the temporal lobe, may be particularly aVected.
Neurobiologic studies of childhood-onset schizophrenia are even fewer in number and have been conducted largely through the NIMH Childhood Onset Schizophrenia Project. This project has involved national recruitment of severely ill, treatment-refractory children and adolescents with well-documented onset of schizophrenia by age 12 (Gordon et al., 1994). Patients in this study undergo a period of drug-free washout to conWrm
diagnosis and to obtain baseline clinical ratings prior to initiation of atypical antipsychotic medication (Kumra et al., 1996, 1999). As a result a high degree of conWdence in the accuracy of the diagnosis is possible with this sample. Furthermore, many of the neurobiologic studies of this sample have been conducted at the end of the medicationfree phase to permit clear separation of medication eVects from disease eVects.
Neurobiologic studies of the NIMH childhood-onset schizophrenia sample have generally supported neurobiologic continuity between childhoodand adult-onset schizophrenia (Jacobsen and Rapoport, 1998). A pattern of abnormalities of eye tracking (Jacobsen et al., 1996), autonomic function (Zahn et al., 1997), and MRI brain morphology, (Jacobsen and Rapoport, 1998) similar to that found in adult-onset schizophrenia has been observed.
Structural brain imaging
Few systematic studies of brain morphology in pediatric schizophrenia have been conducted. One early axial computed tomography (CT) scan study by Schulz and colleagues (1983) of 15 adolescents with schizophrenia or schizophreniform disorder (mean age 16.5"1.55 years; illness duration mean 13 months, range 5±24 months) demonstrated signiWcant increases in ventricular volume in the schizophrenic group relative to both normal controls and a contrast group of borderline patients of similar ages, with greater increases in ventricular/brain ratio being related to poorer treatment response (Schulz et al., 1983). However, using MRI, this group later found no evidence of increased ventricular volume but instead found signiWcantly smaller total brain volume in 17 schizophrenic patients aged 9±18 years (mean 14.3 years) when compared with 13 normal controls (mean age 14.9 years) (Friedman et al., 1996). After correction for group diVerences in total brain volume, there were no diVerences in hippocampal volume (Findling et al., 1996). Yeo and colleagues (1997) studied 20 children with schizophrenia spectrum disorders and 20 healthy children using structural MRI and found decreased amygdala and temporal cortex volumes, but normal hippocampal, ventricular, frontal and total brain volumes.
A recent analysis in which the brain morphology of 28 patients in the NIMH childhood-onset schizophrenia study was compared with that of 57 healthy controls matched for age, sex, and handedness and not diVering in weight or height revealed signiWcantly decreased (by 9.7%) total cerebral volume for the patients with schizophrenia (Jacobsen and Rapoport, 1998), as has been seen in adultonset schizophrenia (Zipursky et al., 1991; Harvey et al.,
1993). Given these large group diVerences, all regional brain comparisons were conducted with analysis of covariance using total cerebral volume as the covariate. As has been observed in adult schizophrenia (Breier et al., 1992; Flaum et al., 1995), the lateral ventricles were increased in volume, and there was a trend for anterior frontal tissue volume (tissue anterior to the coronal plane intersecting the anterior-most point of the corpus callosum) to be decreased in size relative to that in healthy controls. The midsagittal thalamic area was also signiWcantly decreased in patients with childhood-onset schizophrenia. A signiWcant increase in the midsagittal area of the corpus callosum in patients with childhood-onset schizophrenia suggested that sparing of this white matter structure had occurred. In support of this notion, preliminary gray/white segmentation data suggest that the reduction in total cerebral volume in childhood-onset schizophrenia is primarily a consequence of a reduction in gray matter volume, with relative sparing of white matter (Giedd et al., 1996).
A longitudinal MRI brain imaging study of the NIMH childhood-onset schizophrenia sample has shown that, consistent with studies of adult schizophrenia, enlarged basal ganglia volumes on study entry normalize after patients are switched to atypical antipsychotic medication (Frazier et al., 1996). Perhaps most striking, however, has been the observation that the volumes of the lateral ventricles and temporal lobe structures are changing over time during adolescence in the sample more rapidly than in healthy matched controls. The rescanning of 16 patients with childhood-onset schizophrenia and 24 matched yoked controls 2 years following their initial scan indicated that ventricular volume increased more in children with schizophrenia than in healthy children (diagnosis !time F 5 16.1; p 5 0.0003) (Rapoport et al., 1997). In addition, midsagittal thalamic area decreased signiWcantly in schizophrenic patients, but not in controls, over this time period (Rapoport et al., 1997). A rescan after 2 years in 10 patients with childhood-onset schizophrenia and 17 healthy yoked controls showed greater decreases in volumes over time of the right temporal lobe, bilateral superior temporal gyri, and left hippocampus in patients with childhood-onset schizophrenia (Jacobsen et al., 1998). Therefore, although medial temporal lobe structures were not found to be reduced in size in patients with childhood-onset schizophrenia upon their entry into the NIMH study (Jacobsen et al., 1996a), these structures may undergo abnormal developmental changes during adolescence leading to reduced size by early adulthood.
Functional imaging in childhood-onset schizophrenia |
193 |
|
|
|
|
Functional brain imaging: cerebral glucose metabolism and blood Xow
Very few functional brain imaging studies have been conducted in patients with childhood-onset schizophrenia. This fact stems not only from the rarity of this disorder but also from the demands that functional imaging studies place upon subjects. For all functional imaging modalities, it is important to remain still for the duration of the scan to avoid loss or distortion of data from movement artifact. For some modalities, functional imaging also requires performance of cognitive activation tasks, undergoing intravenous and/or arterial line placement, and being oV of antipsychotic medication. Balancing these signiWcant challenges is the important opportunity that functional neuroimaging methodologies provide for testing neurodevelopmental hypotheses of neuropsychiatric disorders.
To the best of our knowledge, only one brief report has appeared in the published literature to date in which cerebral blood Xow (CBF) was examined in pediatric schizophrenia (Chabrol et al., 1986). This study compared 10 neuroleptic-naive adolescents with schizophrenia (mean age 16"1.5, illness duration 1.6"0.7 years) and 10 healthy sexand age-matched controls using xenon-133 as tracer and demonstrated signiWcant hypofrontality in the patients with schizophrenia. The state in which subjects were scanned was not speciWed.
The only study of cerebral glucose metabolism in child- hood-onset schizophrenia has been conducted with a subset of the NIMH childhood-onset schizophrenia sample (Jacobsen et al., 1998). Of particular interest were comparisons between childhoodversus adult-onset schizophrenia. If early-onset schizophrenia is caused by a more pronounced lesion, then one would expect to see greater hypofrontality in patients with childhood-onset disease. To facilitate these comparisons, the methods previously applied to an adult-onset sample, who showed decreased frontal metabolism during the performance of an attentional task (Cohen et al., 1987), were adapted for use with the childhood sample. The adult study examined 16 patients (12 male, four female; mean age 28"7 years) and 27 normal controls (15 male, 12 female; mean age 33"12 years).
The childhood-onset schizophrenia study examined 16 patients (six girls, 10 boys), ages 12 to 18 years (mean"SD 14.2"1.7 years) with a mean age at onset of psychosis of 9.9"1.8 years, using positron emission tomography (PET) and [18F]-Xuorodeoxyglucose (FDG). As in the adult study (Cohen et al., 1987), patients discontinued their medications prior to scanning. Adult patients studied by Cohen were medication free for 13±73 days (mean 34"18) prior to